An official website of the United States government
Official websites use .gov A .gov website belongs to an official government organization in the United States.
Secure .gov websites use HTTPS A lock ( Lock Locked padlock icon ) or https:// means you've safely connected to the .gov website. Share sensitive information only on official, secure websites.
- Publications
- Account settings
- Advanced Search
- Journal List


Development and Plasticity of the Primary Visual Cortex
J sebastian espinosa, michael p stryker.
- Author information
- Copyright and License information
Correspondence: [email protected]
Hubel and Wiesel began the modern study of development and plasticity of primary visual cortex (V1), discovering response properties of cortical neurons that distinguished them from their inputs and that were arranged in a functional architecture. Their findings revealed an early innate period of development and a later critical period of dramatic experience-dependent plasticity. Recent studies have used rodents to benefit from biochemistry and genetics. The roles of spontaneous neural activity and molecular signaling in innate, experience-independent development have been clarified, as have the later roles of visual experience. Plasticity produced by monocular visual deprivation (MD) has been dissected into stages governed by distinct signaling mechanisms, some of whose molecular players are known. Many crucial questions remain, but new tools for perturbing cortical cells and measuring plasticity at the level of changes in connections among identified neurons now exist. The future for the study of V1 to illuminate cortical development and plasticity is bright.
A Legacy of Hubel and Wiesel
The discoveries of Hubel and Wiesel (1962) about V1 fifty years ago laid the ground for much of our current understanding of the development and plasticity of the brain. Three aspects of their approach and findings were crucial. First, they discovered features of neural responses that were distinctly cortical, allowing them to isolate development of the cortex from changes taking place at earlier stages of the nervous system. Second, they focused efforts and explanations not only on a thorough, qualitative understanding of the responses of single neurons but also on hypotheses about the specific neural circuitry that produced these responses. Finally, their investigations of the changes in neuronal responses, which we now refer to as plasticity, were always put in the context of normal and clinically abnormal development. These qualities were evident from the beginning of their work, and they made the visual cortex perhaps the most intensely studied and best understood area of the forebrain for the investigation of development and plasticity.
Distinctive Features of the Responses of Cortical Neurons
Hubel and Wiesel’s initial experiments attempted to stimulate cells in V1 with circular spots of light that were previously shown to be effective in driving neurons in the retina and in the lateral geniculate nucleus, pars dorsalis (LGNd), which provides the major input to V1. Such visual stimuli, however, failed to elicit responses in the majority of neurons in V1. By examining the discharge properties of individual neurons qualitatively and at length, they discovered that neurons in V1 responded to slits or light-dark borders at a specific angle, or “orientation,” and position in the visual field. Most V1 neurons were also binocularly driven, responding to stimulation of either eye, and many were facilitated by stimulating both eyes together. Different neurons responded better to one eye than to the other, and the term “ocular dominance” was coined to refer to the balance between responses to the two eyes. Hubel and Wiesel also observed that neighboring cells in V1 with similar preferred orientations and similar ocular dominance properties were organized in radial columns extending through all the layers of cortex from the surface to white matter ( Figure 1 ; Hubel et al., 1976 ). They referred to this feature of visual cortical organization as “functional architecture.”
Figure 1. Functional Architecture of V1 in Cat and Mouse.
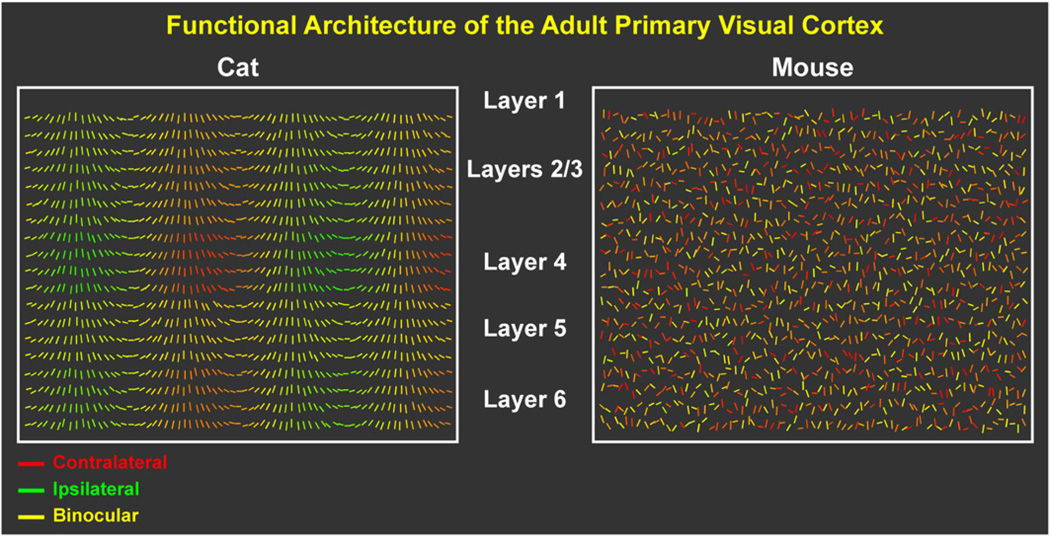
Both cats and mice contain neurons in V1 that must receive and transform precise inputs from the LGNd. V1 in the adult cat (left) consists of neurons highly selective for specific orientations (denoted by the angle of lines) and dominated to varying degrees by the contralateral (red) or ipsilateral (green) eye, with many cells driven by both eyes (yellow). Both orientation and ocular dominance properties are organized into columns. Preferred orientation columns span all cortical layers, while ODCs are most pronounced in layer 4, where many cells are driven monocularly. Mouse V1 (right) does not have columnar organization of orientation or ocular dominance. However, neurons are still highly orientation selective and display a range of ocular dominance but with a bias toward the contralateral eye.
The orientation selectivity and binocularity of neurons are unique properties of V1, entirely absent from the receptive fields of neurons in LGNd, thus making it possible for experimenters to attribute changes strictly to the cortex and to ask fundamental questions about cortical development and plasticity. The other cortical sensory areas do not share such a clear categorical distinction between cortical responses and their inputs because the qualitative responses of cortical cells are like those of cells at lower levels, making inferences about a cortical locus of plasticity more difficult.
Anatomy as the Explanation of Physiology
Hubel and Wiesel were also ahead of their time in attempting to explain the transformation from LGNd to V1 in terms of the connectivity of the underlying circuitry. This focus on anatomy as the explanation for physiology inspired many exciting experiments (reviewed in Reid, 2012 ; Priebe and Ferster, 2012 ), a number of which took advantage of the columnar organization of V1 to interpret the labeling of anatomical connections. Their anatomical interpretation of physiological findings created a bridge between studies of cortex and parallel work in the peripheral nervous system, where the primary tools were in many cases anatomical. Conclusions about the mechanisms of cortical development and plasticity could be reinforced by convergent evidence from anatomical and physiological studies.
Cortical Plasticity
The existence of cortical plasticity had long been appreciated in connection with studies of learning and memory or recovery from injury, but these findings were hard to pursue without a specific understanding of cortical organization and function. Hubel and Wiesel’s work advanced the study of cortical plasticity by putting it firmly in the context of development. Influenced by earlier clinical observations that children with congenital cataracts have permanent visual deficits after removal of their cataracts, Hubel and Wiesel published three papers in 1963 reporting recordings from V1 at different stages in the development of normal kittens and kittens in which the vision of one eye had been occluded by eyelid suture ( Hubel and Wiesel, 1963 ; Wiesel and Hubel, 1963a , 1963b ). Their discovery that MD in kittens during a brief period in early life produced life-long changes in the functional properties of V1 established a model system for the study of cortical plasticity. The requirement that the mechanisms of normal development must organize cortical connections, and that they might be manipulated to do so normally or abnormally, gave a rational framework for the study of plasticity and its mechanisms. These studies also, of course, had profound clinical implications.
The Era of the Mouse
While most of Hubel and Wiesel’s discoveries about V1 were made in cats and monkeys, Dräger and Hubel ( Dräger, 1975 ) and the Pearlman laboratory ( Wagor et al., 1980 ) also pioneered the study of V1 in the mouse 40 years ago, at the time that neurogenetic studies of eye and brain development were beginning to bear fruit and before the modern era of molecular genetics. Recent studies in mouse V1 have demonstrated many similarities with cats and monkeys. For example, the spatial organization of the receptive fields of the most common “simple cells” of mouse V1 appears identical, except for a difference in spatial scale and maximum discharge frequency ( Niell and Stryker, 2008 ).
The functional architecture of V1 does, however, differ ( Figure 1 ). V1 neurons in carnivores and most primates, but not in mice, are arranged in radial columns according to preferred stimulus orientation that progress through a complete cycle of 180 degrees of orientation over about 1 mm of cortex, referred to as an orientation “hypercolumn” ( Hubel et al., 1976 ). The mouse also lacks the much wider ocular dominance columns (ODCs), where neurons favor one eye or the other ( Figure 1 ). In the mouse, neurons selective for different stimulus orientations or for different eyes are scattered throughout V1 apparently at random ( Ohki et al., 2005 ).
Orientation and ODCs made it possible to carry out many important experiments because of the relationship between the location of the neurons and their visual response properties. One could, for example, stimulate or deprive one column of cells and not another and measure the physiology, anatomy, or biochemistry of the cells whose responses were perturbed. In the mouse, one cannot infer visual response properties other than topography from the anatomical location of a neuron; one must measure physiology and anatomy at the level of single cells.
Nevertheless, the precision of receptive field organization in carnivores, primates, and rodents indicates that connections made by neurons in V1 are specific at the level of single cells ( Ko et al., 2011 ). Accordingly, the mechanisms of development and plasticity, which operate at the level of single cells, are thought to be similar or identical, independent of the presence or absence of columnar organization. In this review, we will focus on the studies in whichever species—mouse, rat, ferret, cat, and monkey—best demonstrate the phenomena and mechanisms at issue.
Stages of Development
Development of the V1 neural circuitry takes place in a series of stages, which appear to proceed similarly from mouse to man ( Daw, 1995 ). Different factors guide the establishment of connectivity at the different stages of development ( Figure 2 ).
Figure 2. Timeline of the Development of Mouse V1.
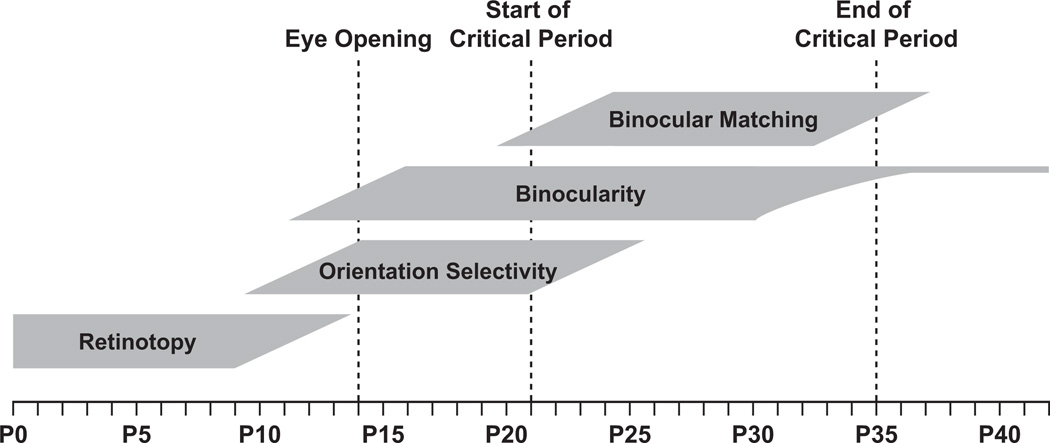
Retinotopic maps form well before eye opening. Orientation-selective and contralateral-eye-driven neurons are present at eye opening. During the subsequent days, neurons become more visually responsive and selective for orientation and respond increasingly well to ipsilateral-eye inputs. At the start of the critical period, individual neurons have mismatched eye-specific preferred orientations. During the critical period this binocular mismatch of orientations is reduced until the end of the critical period when responses reach adult levels.
The first stage we consider in this review is the formation of precise topographic maps. Before the eyes open and before retinal ganglion cells are driven by the rod and cone photoreceptors, axonal projections from the LGNd organize high-resolution point-to-point connections with cells in layer 4 of V1. Experiments discussed below reveal that topographic map formation and refinement is guided by a combination of molecular signaling in the cortex and spontaneous neural activity.
In a second stage of V1 development, orientation selectivity in V1 neurons emerges around the time of eye opening, within days of the first visual responses in the retina. Experiments discussed below reveal that visual experience is not necessary for this stage of development; spontaneous activity suffices.
In the third stage of V1 development, the selective properties of neurons are refined to make them similar through the two eyes. This stage is referred to as the “critical period” because visual deprivation causes rapid and dramatic changes in the strength and organization of inputs from the two eyes to cortical cells. Many experiments described below have characterized the plasticity that can be induced by abnormal visual experience during the critical period and illuminated some of its underlying mechanisms.
Following the critical period, the circuitry and responses of V1 appear mature and normally remain stable throughout life. However, it is still possible for abnormal experience to induce some degree of plasticity in V1 responses and in some of its connections. We discuss below experiments that have characterized adult plasticity and illuminated potential mechanisms that enhance this plasticity.
Experience-Independent Development
Formation of topographic maps.
The mammalian cortex is organized into modality-specific areas that are innervated by their corresponding thalamic nuclei. The initial broad patterning of the cortex into different functionally unique subdivisions, distinguished from one another by their cytoarchitecture and chemoarchitecture, input and output connections, and patterns of gene expression, occurs prenatally in all mammals considered here. Genetic mechanisms involving transcription factors, morphogens, and a number of signaling molecules are responsible for cortical arealization (reviewed in O’Leary et al., 2007 ). If cortical arealization is perturbed by altering the expression of one of these molecules, cortical areas can be enlarged or shrunken, or even duplicated, but the neurons in the resulting altered areas behave identically to those in the normal area of a control animal. Thus, we think of this process as specifying neuronal identity.
After the identity of V1 is established, neurons in V1 are recognized by axons that grow in from the LGNd to form connections within the subplate and later on grow into layer 4 ( Kanold and Luhmann, 2010 ). Neighboring neurons in the retina project their axons to neighboring neurons in the LGNd that, in turn, project to neighboring targets in the V1. Proper function of the visual cortex requires precise, orderly connections from the LGNd to form a single map representing the visual field, allowing neurons in V1 to respond to specific locations in visual space.
The sequence of events and mechanisms involved in the formation of topographic maps in the visual system has been studied most thoroughly in the mouse. The formation of the map of azimuth is guided by a combination of EphA-ephrin-A signaling in the cortex and spontaneous waves of neural activity (reviewed in Feldheim and O’Leary, 2010 ). The EphA family of receptor tyrosine kinases are expressed on the axons of LGNd cells and interact with their ephrin-A ligands that are bound to the surface of neurons in and around V1, where they are expressed in gradients across the representation of the azimuth of the visual field. The mapping of the LGNd projection to V1 was disrupted in ephrin-A2/A3/A5 triple knockout mice or by misexpression of ephrin-A2 or -A5 in V1 ( Cang et al., 2005a ).
During the period of map formation in V1, there are no visual responses because the retinal ganglion cells are not yet driven by the rod and cone photoreceptors. Instead, retinal ganglion cells are excited during this period through cholinergic mechanisms that create waves of ganglion cell discharge that propagate across the retina ( Wong et al., 1993 ). Mice that lack the β2 subunit of the nicotinic acetylcholine receptor (nAChR) or are treated with the cholinergic agonist epibatidine do not have normal retinal waves during the period of map formation and also have disrupted maps in V1 ( Cang et al., 2005b ). In the most dramatic case, disrupting both ephrin-As and cholinergic retinal waves (in ephrin-A2/A5-b2 combination knockout mice) almost completely eliminated the map of azimuth in V1 ( Figure 3 , Cang et al., 2008 ). Surprisingly, the map of elevation was only mildly abnormal, confirming that the two axes of the visual field in V1 are regulated independently; the mechanisms producing the map of elevation are not yet known. Receptive fields of V1 neurons in these mice were elongated in the azimuthal axis, suggesting that V1 neurons are not able to select precise inputs when those inputs are scrambled.
Figure 3. Topographic Azimuth Maps in V1 Depend on EphrinA Signaling and Spontaneous Retinal Activity.
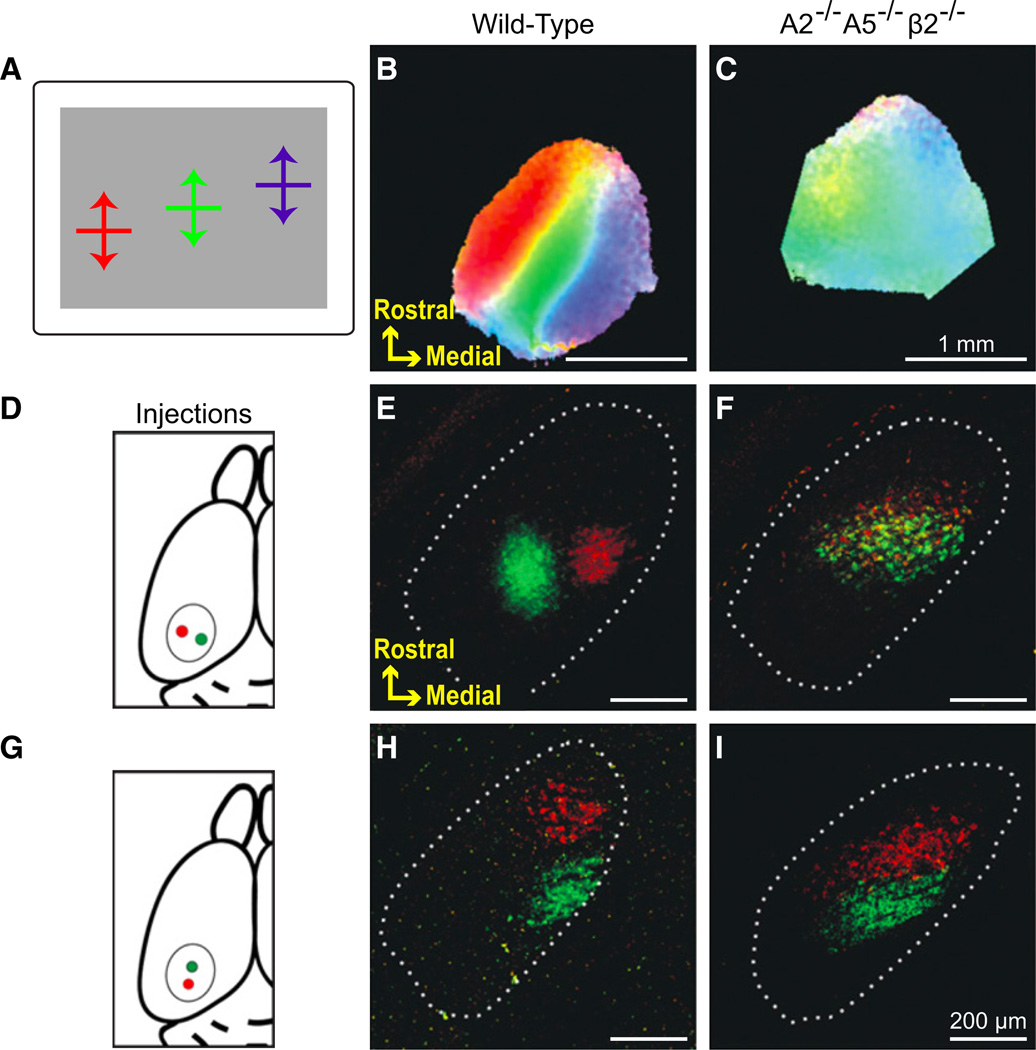
(A) A diagram illustrating spatially restricted stimuli used to assay cortical azimuth maps. The color of each pixel on the cortical maps is determined by the relative response magnitude evoked by the bars along the three positions, with color component according to the diagram.
(B) Wild-type mice with normal azimuth maps.
(C) Combination knockouts that disrupt the majority of EphrinA signaling and early-stage spontaneous retinal activity demonstrate severely disrupted azimuth maps.
(D–I) Neurons in LGNd were retrogradely labeled by injections of CTB-Alexa 488 (green) and CTB-Alexa 568 (red) 500 µm apart in V1 along the lateromedial axis (D). Note the separation between the green and red cells in (E) wild-type, and their complete lateromedial overlap in (F) combination knockouts. Dotted lines mark the border of LGNd. (G) Neurons in LGNd were also retrogradely labeled by injections in V1 along the elevation axis. Note the lack of overlap in (H) wild-type and (I) combination knockouts. Adapted from Cang et al. (2008) .
Development of Selective Receptive Fields and Orientation Columns
After topographic maps have become organized, neurons in V1 acquire inputs in an arrangement that endows them with specific response properties: orientation selectivity ( Priebe and Ferster, 2012 ) and ocular dominance. On the basis of the selective properties of neurons recorded in very young, visually inexperienced cats and neonatal monkeys, Hubel and Wiesel concluded that visual experience was not necessary for the formation of selective receptive fields or the organization of functional architecture, and therefore that “innate” mechanisms determine the organization of receptive fields and cortical columns ( Hubel and Wiesel, 1963 ; Hubel et al., 1976 ). Although this conclusion was called into question by some reports in the following decade, later quantitative studies of single neurons in slightly older animals that had been deprived of light and visual experience from birth confirmed it ( Sherk and Stryker, 1976 ).
Many neurons are selective around the time of natural eye opening, but the responses are typically weaker than in older animals ( Chapman and Stryker, 1993 ; Hubel and Wiesel, 1963 ; White et al., 2001 ; Wiesel and Hubel, 1974 ). Orientation columns Injections are evident at about the same time ( Chapman et al., 1996 ; Crair et al., 1998 ). Binocular visual deprivation by dark-rearing or eyelid suture allows responses to become stronger and more selective for a few weeks as the animal matures ( Crair et al., 1998 ), indicating that most neurons develop selectivity without visual experience. In contrast, blockade of cortical activity by infusion of tetrodotoxin (TTX) prevents the maturation of orientation selectivity ( Chapman and Stryker, 1993 ; White et al., 2001 ). The development of orientation selectivity and orientation columns thus appears to require neural activity in the cortex but is modestly influenced, if at all, by deprivation of visual experience before the beginning of the critical period for ocular dominance plasticity (see below). The earliest appearance of orientation selectivity in V1 might merely reflect sparse inputs; a V1 neuron that is excited by only two inputs will almost certainly respond best to a line that spans the two receptive fields of the inputs. It is still not known whether such initial sparse responses influence the development of mature orientation selectivity ( Ringach, 2007 ).
Some early studies suggested that limiting the visual experience of kittens to contours of a single orientation, parallel black and white stripes of different widths inside the walls of cylinders, caused neurons in V1 to acquire selectivity for the orientation to which the animal had been exposed ( Blakemore and Mitchell, 1973 ), but these results were not confirmed by quantitative measurements of selectivity and additional control procedures ( Stryker and Sherk, 1975 ). A more stringent deprivation procedure using parallel stripes in goggles in which one eye viewed horizontal lines and the other viewed vertical lines for several months revealed that neurons that had received stimulation that matched their innate selectivity remained responsive and selective, while the majority of neurons lost their innate selectivity similar to the effects of prolonged dark-rearing ( Stryker et al., 1978 ). These experiments are consistent with a role for visual experience in the maintenance but not the development of orientation selectivity. However, a recent study in mice provided evidence that the orientation selectivity of some neurons may be altered by rearing with astigmatic lenses that focus a limited range of orientations; while a loss of responsive neurons in the upper half of layer 2/3 could account for the overrepresentation of the experienced orientation there, it could not account for the effects in the lower half ( Kreile et al., 2011 ).
Many neurons in V1 are direction selective as well as orientation selective, but the development and plasticity of direction selectivity is different. Direction selectivity in retinal ganglion cells makes the study of its cortical organization and development difficult, and findings are different among species. In ferrets, direction-preference maps, unlike orientation columns, are absent at eye opening and do not develop in animals reared in darkness, but are highly labile and powerfully influenced by experience with moving visual stimuli ( Li et al., 2008 ). In cats, early experience with stimuli moving in one direction also had long-lasting influences on the direction selectivity of cells in V1 ( Berman and Daw, 1977 ). In mice, direction- as well as orientation-selective neurons were present at eye opening and developed normally even when animals were reared in darkness ( Rochefort et al., 2011 ).
Development of Ocular Dominance
Hubel and Wiesel and their colleagues developed methods to reveal eye-specific segregation of thalamocortical projections that form ODCs in layer 4 of V1. Injection into one eye of transneuronal tracers 3 H-amino acid or sugar reveals bands of dense label in V1 representing that eye’s thalamocortical input ( Wiesel et al., 1974 ). However, this method was not as reliable in young animals because the tracer could leak into inappropriate layers of the LGNd ( LeVay et al., 1978 ).
Using this method, ODCs in monkeys were observed in utero, weeks before the onset of visual experience ( Rakic, 1976 ), and by birth were as precise as in adults ( Horton and Hocking, 1996 ) and clearly functional ( Des Rosiers et al., 1978 ). While the development of ODCs clearly did not require visual experience, the source of the information that allows thalamocortical inputs from the two eyes to segregate was not clear. One possibility is that spontaneous activity is not correlated between the pathways serving the two eyes but is correlated within each eye’s pathway, and that ODC formation, like the formation of topographic maps, is driven by spontaneous activity, which is also present in utero. Another possible source of eye-specific information is hypothetical activity-independent molecular signals from the different layers of the LGNd, perhaps transferred there from the two eyes.
While much of the development of monkey V1 takes place prenatally, similar principles guide V1 development postnatally in cats and ferrets, which are born less mature. In cats, ODCs were not evident using transneuronal labeling before postnatal day (P)7 ( Crair et al., 2001 ). Repeated binocular injections of TTX to block all retinal activity from P14, a few days after eye opening, left thalamocortical afferents unsegregated, and nearly all neurons were driven similarly by the two eyes suggesting that ODCs had failed to form ( Stryker and Harris, 1986 ). Individual thalamocortical afferent arbors failed to withdraw early widespread branches in layer 4 of V1 in such animals ( Antonini and Stryker, 1993a ). Thus, retinal activity blockade either prevents ODCs from forming or desegregates them if they form earlier; the latter suggests that ongoing activity is necessary for maintenance of normal connectivity.
In ferrets, direct injections of anterograde tracers into the developing LGNd, rather than injections of transneuronal tracers into the eyes, showed patchy projections that were interpreted as nascent ODCs 2 weeks before eye opening and before V1 cells were visually responsive, and were present even in animals in which one or both eyes had been removed ( Crowley and Katz, 2000 ). These findings were thought to exclude a role for correlated activity originating in the eyes in the formation of ODCs and suggested that there must be eye-specific molecular labels. However, no LGNd eye-specific layer molecular markers have been discovered to date despite a comprehensive screen ( Kawasaki et al., 2004 ). In addition, there is no evidence that any of the molecules implicated in axon guidance and found in the cortex during ODC formation are involved in column formation ( Dyck and Cynader, 1993 ).
Correlated spontaneous activity might in principle operate to refine patchy thalamocortical connections that might have nothing to do with ocular dominance. Multisite electrode recordings in ferret cortex revealed that correlated spontaneous activity was indeed organized into periodic patterns that might be thought to reflect such early columns ( Chiu and Weliky, 2001 ). In adult animals, waves of activity propagate across the cortex particularly in the absence of a strong stimulus ( Sato et al., 2012 ). Even in the absence of the eyes, spontaneous activity patterns in the LGNd have similar spatial and temporal patterns to those induced by retinal waves ( Weliky and Katz, 1999 ). These sustained bursts in enucleated animals are appropriate for driving activity-dependent segregation of thalamocortical afferents, which depends on large-scale correlations within geniculate laminae ( Miller et al., 1989 ; Willshaw and von der Malsburg, 1976 ). Thus, organized patterns of spontaneous activity in the developing thalamocortical system appear sufficient for column formation, and spontaneous activity originating in the retina is certainly necessary at least for the maintenance of segregated connectivity.
Experience-Dependent Development
After ODCs are formed, responses to the ipsilateral eye remain weaker and less well organized than those to the contralateral eye. Binocular visual deprivation in cats had no effect on the responsiveness or selectivity through either eye until P21, the beginning of the critical period for ocular dominance plasticity (ODP). At that point, the V1 response to the ipsilateral eye became much stronger if the animal was permitted visual experience ( Crair et al., 1998 ). Responses to both eyes deteriorated over the next 3 weeks if binocular deprivation was instituted or continued ( Crair et al., 1998 ), suggesting a powerful role for experience in the maintenance of responsiveness and selectivity. Although mice lack ODCs, individual cells in mouse V1 must still integrate inputs from the two eyes. After eye opening, V1 cells are better driven by inputs from the contralateral eye than those from the ipsilateral eye, and the refinement of ipsilateral eye inputs is influenced by experience-dependent binocular competition ( Smith and Trachtenberg, 2007 ). The emergence of strong ipsilateral responses is not consistent with a purely Hebbian-based model of activity-dependent competition between the two eyes because the stronger contralateral inputs would always outcompete the much weaker ipsilateral inputs. It suggests that some sort of resource-based competition must also be involved ( Kasthuri and Lichtman, 2003 ; Toyoizumi and Miller, 2009 ).
The initial connections to V1 serving the two eyes are organized separately. Before the critical period for ODP, neurons in mice are commonly selective for different orientations when driven through the two eyes ( Wang et al., 2010 ). If there is simultaneous binocular vision during the critical period, the selectivity is gradually altered so that by the end of the critical period the receptive fields in the two eyes come to match, and V1 neurons respond optimally to the same orientation when driven through either eye ( Figure 4 ). Monocular or binocular visual deprivation during the critical period prevented binocular matching, and neurons continued to respond differently through the two eyes throughout life for as long as they have been followed ( Wang et al., 2010 ). These findings reveal a purpose for the critical period in normal development: matching the left eye and right eye receptive fields of V1 binocular neurons.
Figure 4. Emergence of Binocular Inputs and the Matching of Preferred Orientations in Mice.
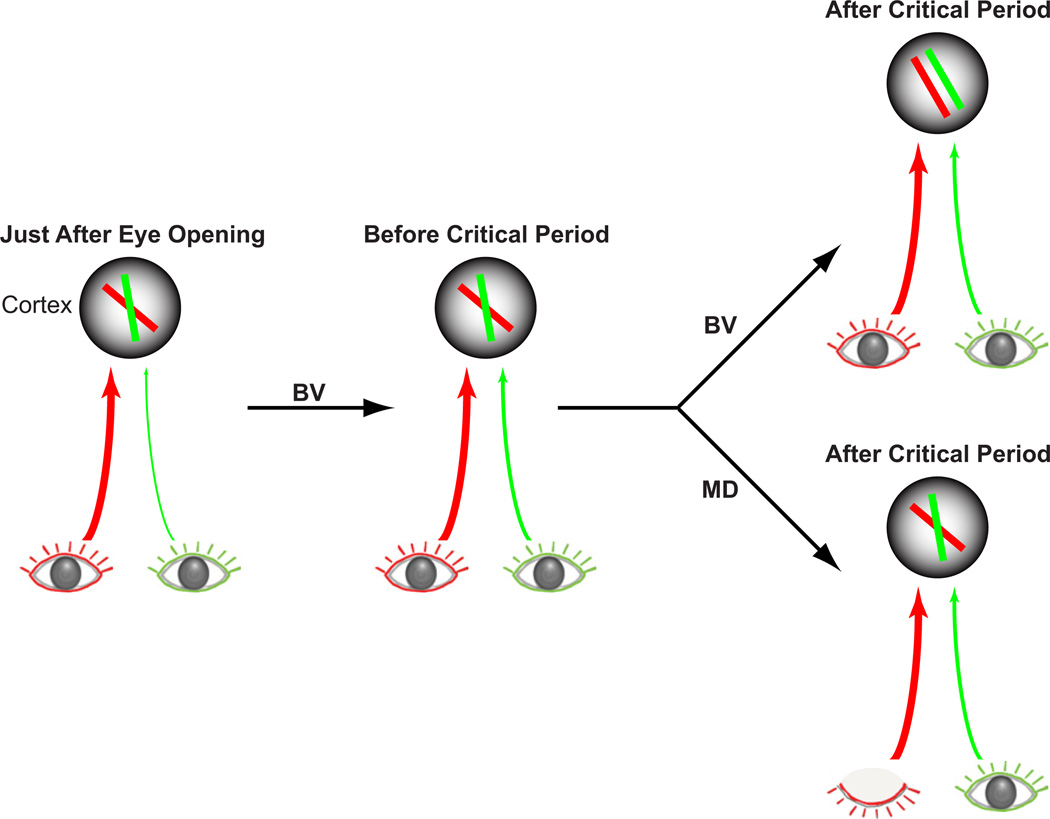
Just after eye opening, individual neurons are selective for specific orientations. The majority of neurons at this point are largely dominated by contralateral-eye (red) inputs. During the next week, before the onset of the critical period, ipsilateral eye (green) inputs strengthen. At this developmental stage, the preferred orientations of individual cortical cells are mismatched through the two eyes. By the end of the critical period, preferred orientations are matched more precisely between the two eyes. Monocular deprivation of visual experience during the critical period permanently blocks the binocular matching of orientation preference. Collectively, normal visual experience during the critical period serves to match eye-specific inputs to individual cortical cells (from Wang et al., 2010 ). BV = binocular vision. MD = monocular deprivation.
The existence of orientation columns in cats makes the corresponding experiment much more difficult to interpret because random connections with other local neurons would still produce an approximate match of orientation. When cats were reared with a reverse suture protocol so that the two eyes were never permitted simultaneous binocular vision but both eyes still drove V1 well, orientation maps elicited through the two eyes continued to match closely ( Gödecke and Bonhoeffer, 1996 ).
Ocular Dominance Plasticity
In 1963, Hubel and Wiesel were the first to illustrate three key points of plasticity induced by MD. First, MD induces a dramatic shift in ocular dominance in V1 and not in earlier processing centers. Second, altered ocular dominance results from competition between inputs from the two eyes. Third, there exists a critical period during development for the plasticity induced by MD. The shift in responses to the two eyes induced by MD in V1 is the best characterized form of ODP.
Hubel and Wiesel’s choice to deprive only one eye of vision allowed them to directly compare the responses of the deprived eye with the nondeprived eye, permitting as an internal control for variations in the level of sedation, health, and developmental stage of the kittens. Monocularly depriving newborn kittens for at least one month induced a dramatic shift in V1 responses from the deprived eye toward the nondeprived eye (83 of 84 cortical cells were unresponsive to the deprived eye) ( Wiesel and Hubel, 1963b ) but had little effect in the LGNd ( Wiesel and Hubel, 1963a ). Notably, merely blurring vision rather than occluding it completely had the same effect in V1 ( Wiesel and Hubel, 1963b ) but no effect on the LGNd ( Wiesel and Hubel, 1963a ).
Hubel and Wiesel hypothesized that the shift in ocular dominance induced by MD results from a competitive loss of deprived-eye connections in the underlying circuitry. This conclusion emerged from their findings in two key experiments. First, young kittens (as young as 8 days) with no previous exposure to patterned stimuli had many cells responding to both eyes similar to those observed in adults, although more sluggishly ( Hubel and Wiesel, 1963 ). Thus, neural connections necessary for visual processing in V1 are already present at or soon after birth. MD from birth could not be explained by a failure of formation of connections—a stark departure from the hypotheses proposed by earlier experiments in dark-reared or binocularly deprived animals ( Riesen, 1961 ). Second, in kittens binocularly deprived from birth for at least 2 months, more than half of the cells continued to respond to both eyes ( Wiesel and Hubel, 1965 ). Since MD for a similar amount of time eliminated almost all deprived-eye responses, Hubel and Wiesel were surprised by this finding, having anticipated that binocular deprivation would wipe out all responses. This then led them to hypothesize that the loss of deprived-eye connections was a result of competition with the nondeprived eye and not simply from disuse.
Responses in V1 were also dramatically changed in kittens whose two eyes received similar levels of sensory input but were kept from working together by alternating occlusion of the two eyes or by inducing divergent strabismus (cutting one of the muscles to each eye so that the two eyes pointed outward instead of straight ahead). Nearly all V1 cells stopped responding to both eyes; instead, each cell was driven by one eye or the other ( Hubel and Wiesel, 1965 ). These findings demonstrated that competition between the two eyes took place at the level of single cells and their inputs, and they stimulated many theoretical studies and models of development ( Stent, 1973 ). They also explained the loss of stereoscopic vision in many patients.
MD in adulthood did not cause the dramatic changes in V1 responses that it did in young animals ( Wiesel and Hubel, 1963b ). By varying the onset and cessation of the deprivation, Hubel and Wiesel were able to define a critical period for ODP induced by MD. During this critical period, between the 4th and 8th weeks of life, just 3–4 days of MD resulted in a dramatic decline in responsive cells and a shift in responses from deprived to nondeprived eye ( Hubel and Wiesel, 1970 ).
Hubel and Wiesel and their colleagues did anatomical tracing experiments to determine whether changes in eye-specific inputs to the cortex might underlie the changes in binocular responses induced by MD. In primates and cats, radioactive tracer injections into one eye not only labeled that eye’s layers of the LGNd but were also transported transneuronally up to the thalamocortical terminals in V1. Following MD in young animals, this method disclosed a contraction of thalamocortical projections serving the deprived eye and complementary expansion of the projections serving the open eye ( Hubel et al., 1977 ).
Anatomical tracing also provided some of the clearest evidence for critical periods of susceptibility to the effects of MD, revealing that certain features of ODP in juvenile animals simply do not take place in older animals, and that different portions of the circuit lose their capacity for plasticity at different times. Long after MD ceased to have effects on thalamocortical projections, it continued to cause changes in the ocular dominance of cortical neurons, suggesting a later critical period for some of the intracortical elements of V1 ( LeVay et al., 1980 ). Their most dramatic examples of different plastic periods for different elements of the circuit were “reverse-suture” experiments on monkeys in which perinatal MD was followed by opening the initially deprived eye and suturing the lid of the eye that was initially open. Initial MD for 3 weeks followed by reverse suture was “most unusual in that it showed completely opposite effects in the two sublaminae” of layer 4C ( LeVay et al., 1980 ). The inputs from the parvocellular layers of the LGNd, which go to the lower part of layer 4C, reflected the second period of MD, after the reverse suture; that is, the patches of layer 4 serving the eye that was open after the reverse suture were expanded, and those serving the other eye were shrunken. The inputs from the magnocellular layers of the LGNd were changed in the opposite direction, reflecting the initial period of deprivation: “It seems that reverse suture [at 3 weeks] came too late to effect any change in the distribution of [magnocellular] afferents” ( LeVay et al., 1980 ). Only in recent years has it become possible to pursue this approach beyond the thalamocortical level to determine whether different elements of the neural circuit within V1 have different critical periods of plasticity.
Following Hubel and Wiesel’s initial discoveries in kittens, ODP induced by MD has been studied widely because the changes are dramatic, reproducible, quantifiable, and restricted to the cortex. The ubiquitous nature of ODP has been demonstrated in rodents ( Domenici et al., 1992 ; Gordon and Stryker, 1996 ), ferrets ( Issa et al., 1999 ), and monkeys ( Horton and Hocking, 1997 ). Although critical periods have been identified in other primary sensory areas, including the auditory ( Chang and Merzenich, 2003 ) and somatosensory cortices ( Fox, 1992 ), the similarity of cortical and subcortical sensory responses in these areas of normal animals makes it less clear to what extent the plasticity observed is strictly cortical. In addition, ODP is taken to be representative of a diverse set of critical periods found in more complex phenomena, such as filial imprinting in nidifugous birds ( Lorenz, 1958 ), acquisition of courtship song in birds ( Brainard and Doupe, 2002 ), auditory localization in barn owls ( Knudsen et al., 2000 ), fear extinction ( Johansen et al., 2011 ), and acquisition of language in humans ( Lenneberg, 1967 ). Moreover, ODP is of clinical significance to the recovery of vision in patients with amblyopia or strabismus ( Hoyt, 2005 ).
More recently the mechanisms of ODP have been studied with the aid of genetics in mice, where the critical period starts after P21 and ends around P35 with peak sensitivity to MD around P28 ( Gordon and Stryker, 1996 ). Despite the lack of ODCs in mice and their significantly lower visual acuity compared to cats and monkeys, many of the functional and anatomical aspects are very similar ( Antonini et al., 1999 ; Niell and Stryker, 2008 ). A number of mechanisms have been proposed to account for the opening of the critical period, the changes induced by MD during the critical period, the smaller, slower and somewhat different changes induced by MD in adults, and the enhancement of adult ODP. We focus on studies that use the most temporally and spatially precise manipulations available and highlight key questions that remain unresolved.
Opening the Critical Period of ODP
Three observations from the rodent visual system suggest that the function of particular inhibitory neurons is important for opening the critical period. First, an adequate level of inhibition by the neurotransmitter γ-aminobutyric acid (GABA) is necessary. Second, GABAergic transmission via α1 subunit containing GABA A receptors is necessary. Third, factors that open a critical period also promote the maturation of inhibitory circuits.
Potent GABAergic inhibition is necessary to open the critical period, and a transient enhancement of inhibition is sufficient to open it precociously. GABA is synthesized by two isoforms of glutamic acid decarboxylase, weighing 65 kDa (Gad65) and 67 kDa (Gad67). Gad67-knockout mice are embryonic lethal ( Asada et al., 1997 ), but Gad65-knockout mice are viable and have reduced GABA release in response to stimulation ( Hensch et al., 1998 ). They developed normal baseline receptive field properties in V1, but brief MD had no effect: the critical period of ODP never opened ( Hensch et al., 1998 ). Enhancing inhibition by infusing diazepam (an agonist of the GABA A receptor that increases inhibitory conductance when GABA binds) into V1 restored ODP. Brief administration of diazepam at any age could open a period of susceptibility to the effects of MD in Gad65-knockout mice that was similar in quality and duration to the normal critical period ( Fagiolini and Hensch, 2000 ). Subsequent administrations of diazepam could not open a second critical period. Remarkably, diazepam treatment in wild-type mice at P15, before the normal critical period, could also initiate a single precocious critical period with a similar 2 week duration ( Fagiolini and Hensch, 2000 ). This finding suggests that a transient increase in GABAergic transmission is sufficient to open the critical period using machinery that is already in place earlier in development. Opening the critical period appears to trigger unknown mechanisms that lead to its permanent closure 2 weeks later.
Subsequent studies narrowed the requirement of GABAergic transmission for the opening of the critical period of ODP to the GABA A receptors containing the α1 subunit. Diazepam binds to several GABA A receptor subtypes, including α1, α2, α3, α5, and γ2 ( Sieghart, 1995 ). Using knockin mice with diazepam-insensitive GABA A receptor subunits, Fagiolini et al. (2004) demonstrated that mutant α2 or α3 GABA A receptor subunits, but not α1 subunits, could still produce a precocious critical period, as in wild-type mice, when diazepam was administered. This experiment suggests that inhibitory neurons like the parvalbumin-expressing (PV) basket cells, which make contacts onto GABA receptors containing the α1 subunit, may play a special role in opening the critical period, although it remains possible that inputs onto receptors containing α5 and γ2 subunits may also be necessary.
In normal development, the maturation of the underlying inhibitory circuitry appears to be important for opening the critical period. Several molecular factors that regulate the opening of the critical period also regulate the development of inhibitory neurons in V1. Transgenic animals overexpressing brain-derived neurotrophic factor (BDNF) in excitatory neurons had a precocious critical period and accelerated development of high visual acuity; they also had earlier maturation of inhibitory neurons ( Hanover et al., 1999 ; Huang et al., 1999 ). Other studies suggest roles for polysialic acid neural cell adhesion molecule (PSANCAM), the homeoprotein transcription factor, orthodenticle homolog 2 (Otx2), and IGF-1 in both the opening of the critical period and the maturation of inhibitory innervation, specifically the perisomatic contacts by PV basket cells onto pyramidal cells ( Ciucci et al., 2007 ; Di Cristo et al., 2007 ; Sugiyama et al., 2008 ). The maturation of inhibitory circuits may be responsible for the opening of the critical period merely because of an increase in overall inhibition. Alternatively, inhibitory maturation may produce a pattern of activity or a reconfiguration of cortical circuitry that opens the critical period independent of the level of inhibition.
The onset of the critical period also depends on visual experience. Raising animals in the dark or depriving them of binocular vision from birth delays the opening of ODP induced by monocular visual experience ( Iwai et al., 2003 ). Dark-reared mice exhibit a reduction in BDNF levels ( Zafra et al., 1990 ) and in GABA-mediated transmission ( Morales et al., 2002 ), and the delayed opening of a period of plasticity can be abolished by BDNF overexpression ( Gianfranceschi et al., 2003 ) or direct diazepam infusion ( Iwai et al., 2003 ). These findings suggest that the effects of dark-rearing on plasticity also involve the maturation of inhibitory function as discussed above. However, it is important to note that the plasticity induced by monocular visual experience after dark-rearing is distinct from conventional ODP induced by MD. Conventional ODP operates to alter the function of a V1 circuit that is fully responsive and selective. Dark-rearing causes many neurons in V1 to lose selectivity and become poorly responsive ( Wiesel and Hubel, 1965 ). Thus, the circuit that serves as the substrate for plasticity induced by monocular visual experience after dark-rearing is abnormal. Moreover, dark-rearing also affects the refinement of circuits in earlier visual processing centers, such as the retina ( Tian and Copenhagen, 2003 ) and LGNd ( Akerman et al., 2002 ). Additionally, opening the eye after dark-rearing to measure ODP likely invokes molecular mechanisms that are common to normal eye opening and not shared in the closing of one eye ( Gandhi et al., 2005 ). For these reasons, it is inappropriate to refer to dark-rearing as merely delaying the critical period of ODP.
Perturbation experiments that alter the timing of the critical period generally have not established whether the altered critical period shares all the features of the normal one. An early- or late-onset critical period may lack some of the refinement of visual responses that takes place during the normal one, such as the binocular matching of orientation preferences ( Wang et al., 2010 ).
While the studies discussed above suggest that the rate-limiting step for opening the critical period is the maturation of inhibitory function, other unexplored circuits may also be necessary and sufficient. For instance, maturation of inhibition may affect V1 network activity and open the critical period by promoting fidelity in the temporal structure of excitatory activity ( Wehr and Zador, 2003 ) or by homeostatically increasing overall excitation ( Turrigiano and Nelson, 2004 ). Thus, it would be informative to explore how excitatory and neuromodulatory circuits in V1 mature during the normal opening of the critical period and how they are affected by manipulations that shift the timing of the opening.
These studies provide clear evidence that the critical period, regardless of what triggers its onset, stays open for a limited duration of approximately 2 weeks. It is unclear what changes in the activity, biochemistry, or structure of the V1 circuit renders it no longer as susceptible to MD. Progress will depend on an understanding of how V1 is different at the end of the critical period than at its beginning, even with normal visual experience. For example, now that we understand that binocular matching of orientation selectivity progresses during the critical period ( Wang et al., 2010 ), it appears possible that the attainment of binocular matching itself could prevent further effects of MD. After the critical period, when inputs from the two eyes produce the same pattern of responses in V1 neurons, activity through the open eye may sustain the connections serving both eyes during MD. In contrast, before or during the critical period, when inputs from the two eyes to a particular V1 neuron are driven by different stimuli rather than coherently, they may compete, and the deprived eye would lose out.
The Stages of Critical Period ODP
The use of mice as a model system allowed the development of methods to measure visual responses to the two eyes in V1 repeatedly in individual animals. Transcranial optical imaging of intrinsic signals ( Bonhoeffer and Grinvald, 1996 ) and chronic implantation of recording electrodes to measure the amplitude of visually evoked potentials (VEPs) both allow repeated sampling of the same brain region before, during, and after manipulations of visual experience ( Kaneko et al., 2008a ; Sawtell et al., 2003 ). Both allow reproducible measures of the magnitudes of the separate deprived- and nondeprived-eye responses. Optical imaging through an intact skull has the advantage of being noninvasive, but it is done in anesthesized animals ( Kaneko et al., 2008a ). VEPs have the advantage that they are commonly done in awake mice, but require precise and stable electrode placement ( Sawtell et al., 2003 ) and the amplitude of VEPs are susceptible to change with repeated presentations of grating stimuli of a single orientation ( Frenkel et al., 2006 ). An alternative approach can use VEPs to measure absolute visual acuity ( Fagiolini et al., 1994 ). Neither optical imaging nor VEPs measure the selective responses of single neurons directly.
The methods above were used to dissect ODP induced by MD during the critical period into temporally distinct stages ( Figure 5 ). In the first stage, 2–3 days of MD caused a large reduction of the response to the deprived eye and a resulting shift in ocular dominance, with no change in open-eye responses. In the second stage, MD caused a large increase in the response to the open eye, along with a slight increase in deprived-eye responses, completing the shift in ocular dominance ( Kaneko et al., 2008b ). Restoring binocular vision by reopening the deprived eye during the critical period induced a third stage of plasticity, the rapid restoration of both eyes’ responses to baseline levels ( Kaneko et al., 2008a ). These three stages and their characteristics were similar regardless of which eye was deprived, contralateral or ipsilateral eye ( Sato and Stryker, 2008 ). Collectively, these findings in the mouse are consistent with observations in other species that a decrease in deprived-eye responses precedes an increase in nondeprived-eye responses ( Mioche and Singer, 1989 and references therein).
Figure 5. Stages of Critical Period ODP in Mice.
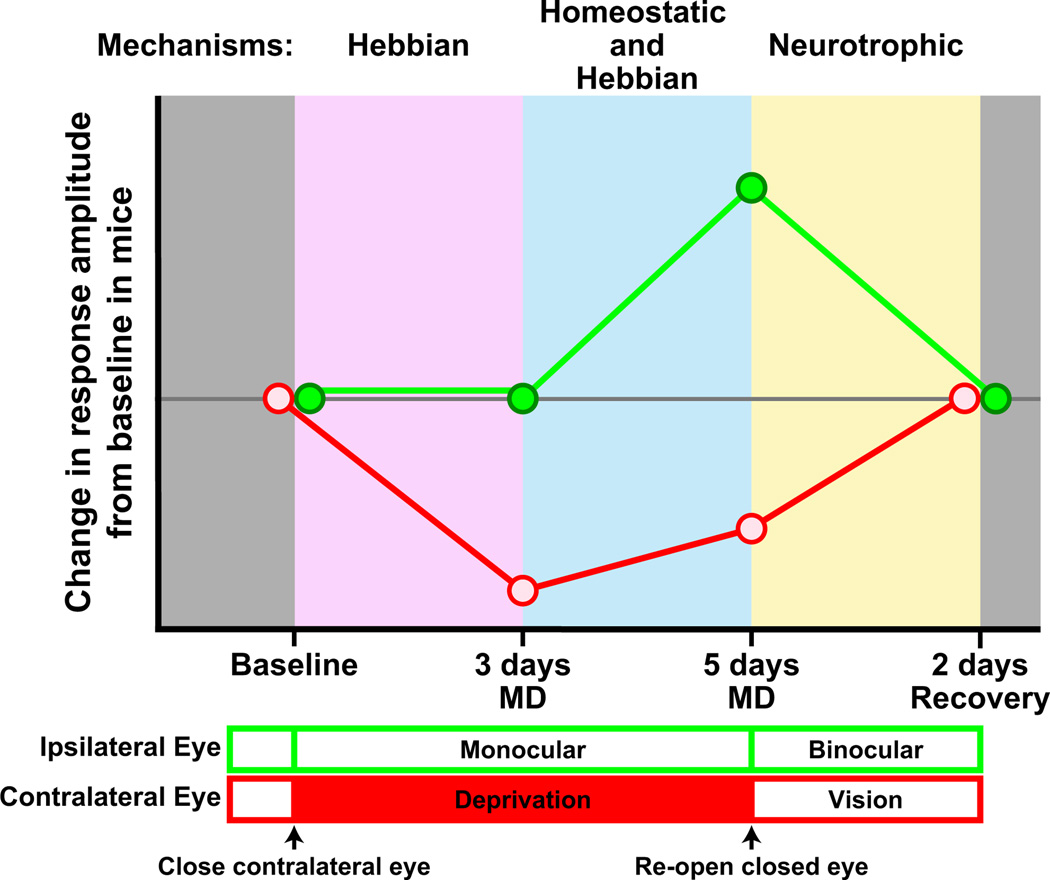
ODP induced by MD during the critical period in mice is characterized by three temporally and mechanistically distinct stages: (1) a Hebbian-dependent dramatic loss of response to the deprived eye (red) after 2–3 days of MD, (2) a Hebbian and homeostatic-dependent increase in open-eye (green) response together with a slight increase in deprived-eye response after 5 days of MD, and (3) a neurotrophic signaling-dependent return of responses to baseline levels after reopening the deprived eye and restoring binocular vision.

The Mechanisms of Critical Period ODP
In cats, pharmacological perturbations confined to V1, such as hyperexcitation by glutamate ( Shaw and Cynader, 1984 ) or bicuculline ( Ramoa et al., 1988 ) or total silencing by TTX ( Reiter et al., 1986 ), 2-amino-5-phosphonovaleric acid (APV) ( Bear et al., 1990 ), or muscimol ( Reiter and Stryker, 1988 ), revealed that neural activity in V1 plays a critical role in ODP. The past decade has seen the creation of transgenic mice in which critical period timing and the development of response properties are normal, but the changes in responses and circuitry during critical period ODP are perturbed. These studies reveal that the three stages of critical period ODP expression are mechanistically distinct ( Figure 5 ): (1) The initial reduction in deprived-eye responses relies on a mechanism involving calcium signaling with pharmacology similar to long-term depression (LTD). (2) The later increase in open-eye responses involves both homeostatic and long-term potentiation (LTP)-like mechanisms. (3) The restoration of normal visual responses after opening the deprived eye involves neurotrophic signaling mechanisms.
The first stage of critical period ODP , the decrease in deprived-eye responses, is hypothesized to result from a loss of deprived-eye connections or a depression in their synaptic efficacy. Consistent with this idea, blocking ( Bear et al., 1990 ) or genetically deleting N-methyl-D-aspartate receptors (NMDARs) ( Roberts et al., 1998 ), manipulations that block LTD, also prevented a shift in ocular dominance. However, these manipulations can also affect LTP and other forms of plasticity. Viral expression of a peptide that blocks LTD and, specifically, NMDAR-dependent internalization of postsynaptic α-amino-3-hydroxy-5-methyl-4-isoxazolepropionic acid receptors (AMPARs) also blocked the reduction in deprived-eye responses in layer 4, consistent with the operation of LTD in the first stage of critical period ODP ( Yoon et al., 2009 ).
Spike timing-dependent plasticity (STDP) is an alternative mechanism that shares a dependence on NMDARs and calcium signaling and appears, at least in the short term, to be a potential explanation of changes during MD ( Yao and Dan, 2005 ). STDP has the advantage that it can either increase or decrease the strength of a connection by altering the timing of action potentials in the two connected cells, without requiring the firing rate changes needed to shift from LTD to LTP. This feature makes it particularly attractive in accounting for the effects of strabismus, where two pathways can be equally active but are not correlated. It is not yet clear what signaling mechanisms would dissociate STDP from LTD/LTP or other forms of plasticity.
Calcium influx through NMDARs ( Daw et al., 1993 ) triggers downstream effectors including protein kinases and phosphatases that are hypothesized to regulate ODP by controlling phosphorylation of substrates thought to be important for synaptic transmission, neuronal excitability, and morphological stabilization: RII-α and RII-β isoforms of cAMP-dependent protein kinase (PKA) ( Fischer et al., 2004 ; Rao et al., 2004 ), extracellular-signal-regulated kinase (ERK) ( Di Cristo et al., 2001 ), α-calcium/calmodulin-dependent protein kinase II (αCaMKII) ( Taha et al., 2002 ), and the phosphatase calcineurin ( Yang et al., 2005 ). In all cases, preventing the activation of the kinases or promoting the activation of the phosphatase prevented the reduction in deprived-eye responses. Collectively, these studies suggest that the balance between protein kinases and phosphatases is important for critical period ODP.
The activity-dependent immediate early gene Arc is a potential mediator of protein synthesis-dependent plasticity. Arc gene expression and efficient Arc translation are dependent on NMDAR and group 1 metabotropic glutamate receptor (mGluR) activation ( Steward and Worley, 2001 ). In Arc-knockout mice, 3 days of MD failed to reduce deprived-eye responses ( McCurry et al., 2010 ). Another activity-dependent immediate early gene, serine protease tissue plasminogen activator (tPA), increases during MD in V1 and targets many downstream effectors including extracellular-matrix proteins, growth factors, membrane receptors, and cell-adhesion molecules ( Mataga et al., 2002 and references therein). In tPA-knockout mice critical period ODP was impaired and could be rescued by exogenous tPA ( Mataga et al., 2002 ). MicroRNAs induced by visual experience may also play a role in ODP. Increasing ( Tognini et al., 2011 ) or decreasing ( Mellios et al., 2011 ) the levels of a microRNA enriched in the brain (miR132), reduced critical period ODP and had dramatic effects on spine morphology.
It is not yet clear to what extent the changes in visual responses in vivo during ODP are the product of changes in the anatomical circuits, such as loss of synapses serving the deprived eye, or changes in synaptic efficacy, such as LTD, within stable anatomical circuits. Figure 6 illustrates this distinction for the first phase of ODP. In terms of biochemical mechanisms the distinction is whether there is a single pathway leading from changes in synaptic efficacy to mechanisms of regulation of growth and retraction or whether, instead, there are parallel and independent processes regulating synaptic efficacy and anatomical change, allowing for the possibility of blocking one without the other. Additional knowledge of pruning mechanisms regulating anatomical changes may allow this distinction to be tested experimentally ( Li and Sheng, 2012 ).
Figure 6. Possible Mechanisms for the Loss of Deprived-Eye Responses during the First Stage of Critical Period ODP.
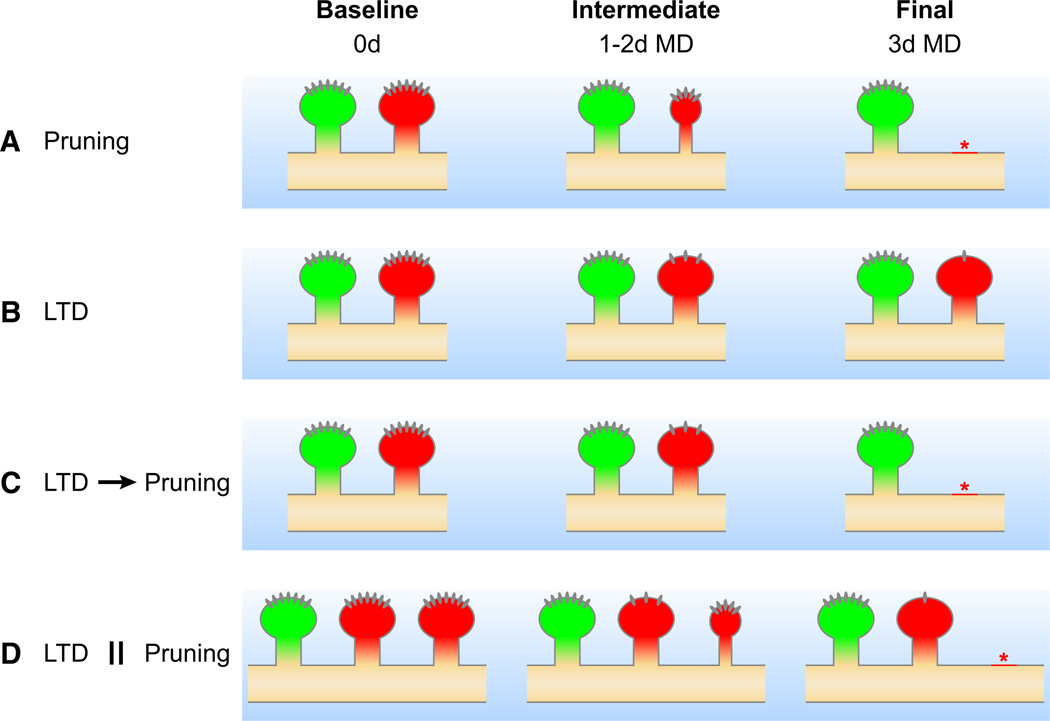
(A) The reduction of deprived-eye responses after 3 days of MD results solely from the selective anatomical pruning of deprived-eye connections, shown here as the disappearance of spines labeled in red.
(B) The depression of deprived-eye responses results solely from the reduction in synaptic efficacy of deprived-eye connections by LTD-like mechanisms that last for days, shown here as the progressive removal of ionotropic receptors from spines labeled in red.
(C) LTD causes the rapid removal of ionotropic receptors and then triggers slower mechanisms that prune the deprived-eye connections that had been rendered ineffective by the removal of receptors.
(D) Pruning and LTD are independently triggered and act in parallel to reduce responses to the deprived eye.
In all cases (A–D), nondeprived-eye connections are unchanged, shown here as spines labeled in green. Longitudinal imaging of structure coupled with temporally defined perturbations that selectively disrupt changes in synaptic efficacy or anatomy would resolve the primary mechanism involved in the first stage of ODP. For example, selectively blocking anatomical changes could inhibit (A or C), partially inhibit (D), or have no effect (B) on ODP.
Assuming that protein synthesis is required for structural changes, Taha and Stryker (2002) attempted to distinguish between these alternatives by blocking it. Protein synthesis inhibitors in the cortex, but not in the LGNd, completely prevented ODP. This result suggested that anatomical plasticity is necessary for ODP, but it left open the possibility that protein synthesis inhibition had also interfered with changes in synaptic efficacy. LTD is conventionally divided into a late phase that is dependent on protein synthesis and an early phase that is not ( Kauderer and Kandel, 2000 ). Thus, the protein synthesis independent early phase of LTD contributes little or nothing to ODP.
The second stage of critical period ODP , the increase of open-eye responses, was difficult to study mechanistically because manipulations that prevent the reduction of deprived-eye responses also affect subsequent increases in the open-eye responses. A two-photon calcium imaging study showing that MD actually increased responses to the deprived eye in neurons with little to no input from the open eye suggested that Hebbian mechanisms were not involved in the second stage of ODP ( Mrsic-Flogel et al., 2007 ) and that homeostatic scaling may operate to keep neural activity within an optimal range ( Turrigiano and Nelson, 2004 ). Mice deficient for tumor necrosis factor-alpha (TNFα), a protein necessary for homeostatic scaling of excitatory and inhibitory synapses ( Stellwagen and Malenka, 2006 ), allowed the dissociation of the first and second stages of ODP and identification of a homeostatic mechanism involved in the second stage. In TNFα-knockout mice, the first stage of ODP was completely normal but there was no subsequent increase in the open-eye responses measured by intrinsic signal imaging; similar results were found in wild-type mice with blockade of TNF receptors in the cortex ( Kaneko et al., 2008b ). Antagonizing NMDARs in wild-type mice using 3-(2-carboxypiperazin- 4-yl)-propyl-1-phosphonic acid (CPP) during the second stage of ODP also prevented an increase in open-eye responses measured by VEPs in layer 4 ( Cho et al., 2009 ). Taken together, these findings indicate that homeostatic as well as LTP-like mechanisms are important for the second stage of ODP.
The third stage of critical period ODP , the restoration of responses to baseline levels following the reopening of the deprived eye, is dependent on neurotrophic growth signaling mechanisms. Previous experiments hypothesized that ODP resulted from competition for limiting amounts of the activity-dependent neurotrophin, BDNF (reviewed in Bonhoeffer, 1996 ). The deprived-eye pathway was thought to lose out to the open-eye pathway because of its failure to stimulate sufficient BDNF release onto its TrkB receptor. This hypothesis was tested in mice using a chemical-genetic approach, the Shokat inhibitor, that gives both high molecular and temporal specificity ( Chen et al., 2005 ). Specifically blocking TrKB receptor activation during the first and second stages of ODP had no effect. Instead, TrkB receptor activation was required for the recovery of both deprived- and nondeprived-eye responses after restoration of binocular vision ( Kaneko et al., 2008a ). Interestingly, the BDNF that mediates recovery appears to be synthesized in dendrites ( Kaneko et al., 2012 ). Consistent with these findings, BDNF levels decrease during MD and return to normal levels after the restoration of binocular vision. Taken together, BDNF-TrkB signaling is not important for the loss of connections but is important in facilitating the growth or strengthening of connections, presumably those of the deprived-eye circuits, to bring back the balance of inputs from both eyes ( Figure 7 ).
Figure 7. Hypothesized Model for Structural and Functional Plasticity in V1.
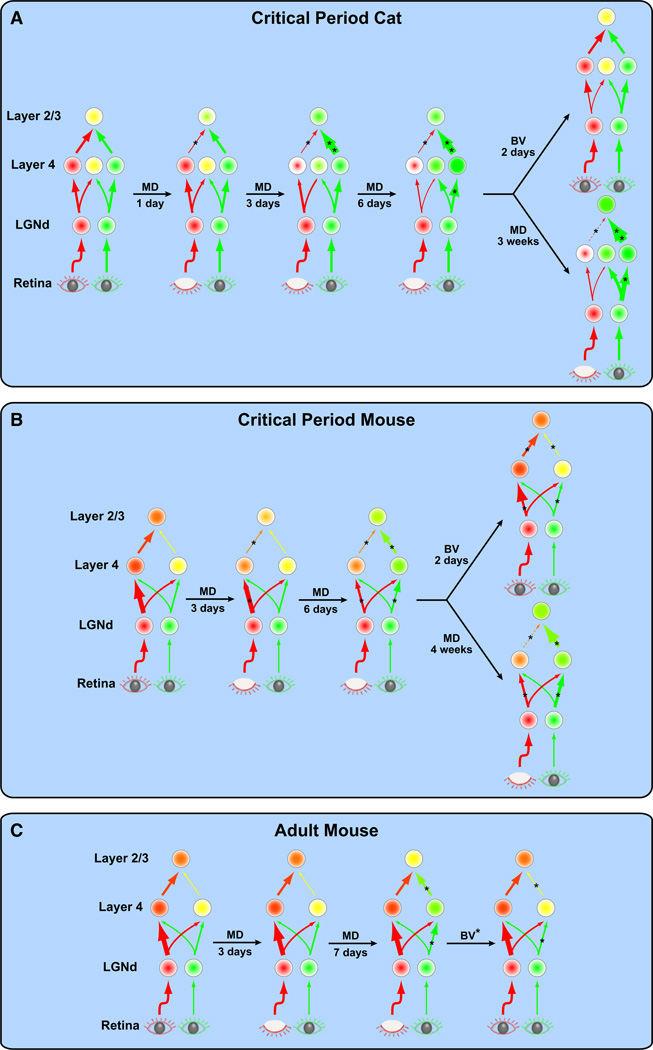
ODP induced by MD in cats and mice has little to no effect on eye-specific projections to the LGNd, but has dramatic effects on cortical function and structure. Diagrams in (A)–(C) depict changes occurring in visual centers in one hemisphere of the brain. Each circle represents a population of neurons. The color and intensity within each circle indicates the response level to the contralateral (red) or ipsilateral (green) eye with binocular responses shown as a mixture of red and green (yellow). Arrows represent information flow between centers of the visual pathway. The size of the arrowhead is proportional to the level of activity. The thickness of the arrow is proportional to the number of connections. Asterisks (*) indicate hypothesized changes that have not yet been experimentally measured.
(A) In critical period cats, changes in cortical responses and connections occur faster in upper cortical layers than in cortical layer 4. Moreover, changes in deprived-eye responses and anatomy precede those of the nondeprived eye. Just 1 day of MD results in a reduction of deprived-eye responses and connections in upper cortical layers, but no change in layer 4. After 3 days of MD, deprived-eye responses are now reduced in layer 4 without accompanying structural changes. In addition, there is an increase in nondeprived-eye responses and connections in upper cortical layers, but not layer 4. MD for 6 days produces no further changes in upper cortical layers. However, in layer 4, deprived-eye connections are lost and now accompany the earlier reduction in deprived-eye responses. Additionally, nondeprived-eye responses in layer 4 get stronger, but without accompanying structural changes. Prolonged MD (3 weeks) results in further reduction of deprived-eye responses and connections in upper cortical layers. Nondeprived-eye responses are further increased in upper cortical layers and nondeprived-eye connections are increased in all cortical layers. Alternatively, reopening the deprived eye after brief MD for just 2 days restores responses to baseline levels in all cortical layers and restores connections in upper cortical layers, but is not sufficient to restore layer 4 connections.
(B) In critical period mice, changes induced by MD occur slower than in critical period cats, but follow a similar progression. After 3 days of MD, deprived-eye responses and connections are reduced in upper cortical layers. In addition, deprived-eye responses are reduced in layer 4, although to a lesser extent and with little or no structural change. After 6 days of MD, there is an increase in nondeprived-eye responses and connections in upper cortical layers. Deprived-eye connections are also lost in layer 4 and now accompany the earlier reduction in deprived-eye responses. Non-deprived-eye responses in layer 4 also get stronger, but without accompanying structural changes. Prolonged MD (4 weeks), results in further reduction of deprived-eye responses and connections in upper cortical layers. Non-deprived-eye responses are further increased in upper cortical layers and connections are increased in all cortical layers. Alternatively, reopening the deprived eye after brief MD for just 2 days restores responses to baseline levels in all cortical layers and restores connections in upper cortical layers, but is not sufficient to restore layer 4 connections.
(C) In adult mice, MD induces qualitatively and quantitatively different changes compared to critical period mice. After 3 days of MD, there are no changes in eye-specific responses or connections. After 7 days of MD, there is an increase in nondeprived-eye responses and connections in upper cortical layers. Nondeprived-eye responses also get stronger in layer 4, but without accompanying structural changes. There are no changes in deprived-eye responses or connections across all layers throughout this period of MD. After reopening the deprived eye, nondeprived-eye responses and connections are restored to baseline levels in all cortical layers.
MD = monocular deprivation. BV = binocular vision.
Enhancement of Critical Period ODP
Several studies have also shown that critical period ODP can be enhanced or accelerated. Mutant mice lacking the paired-immunoglobulin- like receptor B (PirB), a major histocompatibility complex class I (MHC1) receptor, or mice lacking cell surface expression of 2 of the 50+ MHC1 genes, H2-K b and H2-D b , had a larger or faster ocular dominance shift ( Datwani et al., 2009 ; Syken et al., 2006 ). More recently, Kaneko et al. (2010) found that all the stages of ODP were accelerated in mice expressing a constitutively active form of H-ras (H-ras G12V ) presynaptically in excitatory neurons. Measurements in vitro showing enhanced presynaptic facilitation in the connections from layer 4 to layer 2/3 provided a potential explanation for the increased rate of plasticity. Future genetic gain-of-function strategies like those conducted for H-Ras may identify specific molecules that can enhance specific stages of critical period ODP.
The Stages of Adult ODP
The classical studies by Hubel and Wiesel characterizing the time course of MD in cats ( Hubel and Wiesel, 1970 ) and in monkeys ( Hubel et al., 1977 ; LeVay et al., 1980 ) led to the notion of a critical period for ODP in V1 that ends around the onset of adolescence. While it has been well established in numerous species that ODP is most readily elicited byMD early in postnatal development, and thalamocortical afferent anatomy ceases to change, detailed analysis in cats and rats showed that ODP of cortical responses tapers slowly and can linger well beyond sexual maturity ( Daw et al., 1992 ; Guire et al., 1999 ). Similarly, mouse V1 does not abruptly lose its capacity for ODP at the end of the critical period, but instead plasticity declines progressively to an insignificant level by P110 ( Lehmann and Löwel, 2008 ). The characteristics of plasticity also change with circuit maturation from critical period to adult. Lesions and other manipulations have demonstrated substantial plasticity in responses and connections in adult V1 ( Gilbert and Li, 2012 ).
In adult (P60–90) mice, ODP is quantitatively and qualitatively different from critical period ODP in five respects. First, a longer contralateral MD is necessary to induce an observable shift in ocular dominance ( Sato and Stryker, 2010 ; Sawtell et al., 2003 ). Even after 7 days of MD, the ocular dominance shift is less than that found in critical period mice with 4 day MD. Second, the shift in ocular dominance in adults induced by contralateral MD is predominantly an increase in open-eye responses with only a small and transient decrease in deprived-eye responses ( Hofer et al., 2006 ; Sato and Stryker, 2008 ; Sawtell et al., 2003 ). Third, ipsilateral deprivation in adult mice produces no significant ODP ( Sato and Stryker, 2008 ). Fourth, binocular deprivation in adult mice results in a substantial ocular dominance shift ( Sato and Stryker, 2008 ). Fifth, adult ODP is less permanent than critical period ODP, with recovery after restoration of binocular vision taking half as long after long-term MD ( Prusky and Douglas, 2003 ). While ODP in young adult mice clearly differs from that in the critical period, the decline of plasticity in older adults suggests that plasticity mechanisms may continue to change later in life.
The Mechanisms of Adult ODP
Relatively little is known about the molecular mechanisms of adult ODP in the mouse and the extent to which they are similar to those that operate in the critical period. Some mechanisms, such as dependence on calcium signaling through NMDARs, are shared. Adult mice treated with the competitive NMDAR antagonist, CPP, or mice lacking the obligatory NMDAR subunit, NR1, in cortex exhibited no adult ODP ( Sato and Stryker, 2008 ; Sawtell et al., 2003 ). Other mechanisms of critical period ODP are not shared with adult ODP. For instance, adult TNFα-knockout mice that lack homeostatic scaling in vitro had normal increases in open-eye responses following MD while adult αCaMKII;T286A mice, which have a point mutation that prevents autophosphorylation of αCaMKII, lacked the strengthening of open-eye responses following MD ( Ranson et al., 2012 ). Further evaluation of the shared and distinct molecular mechanisms between critical period and adult ODP may reveal the factors that account for the decline in plasticity with age.
Closure of the Critical Period and Adult ODP
The decline of ODP after the critical period may require “brakes” on plasticity mediated by specific molecular mechanisms to close the critical period and their continuous application to keep it closed (reviewed in Bavelier et al., 2010 ). There is evidence for several such mechanisms: persistently potent inhibition, neuromodulatory desensitization, and an increase in structural factors that inhibit neurite remodeling. Below we discuss some of the studies that have taken genetic and pharmacological approaches to interfere with these mechanisms in order to restore juvenile forms and levels of plasticity to adult V1. Although these studies clearly demonstrate that adult ODP can be enhanced, it is unclear whether the approaches used open a new critical period, facilitate existing adult mechanisms, or induce ectopic mechanisms.
Diazepam treatment in adult Gad65-knockout mice or early in development in wild-type mice is capable of opening only one critical period of ODP ( Fagiolini and Hensch, 2000 ). Once the critical period is opened, inhibitory drive increases. This increased inhibition may also be responsible for closing the critical period and keeping it closed. An increased rate and magnitude of ODP following infusion of GABA A antagonist picrotoxin (PTX) or the GABA synthesis inhibitor 3-mercaptopropionic acid (3-MPA) into V1 of adult rats provided partial confirmation of the hypothesis that reducing inhibitory drive in adulthood could enhance ODP ( Harauzov et al., 2010 ).
Studies using lesions and pharmacology in young cats suggested that a combination of cholinergic and noradrenergic transmission was necessary for critical period ODP induced by MD ( Bear and Singer, 1986 ), leading to the hypothesis that a reduction in transmission of either neuromodulator could force the closure of the critical period and prevent ODP. Knockout of an endogenous prototoxin, Lynx1, which reduces cholinergic transmission during adulthood, enhanced adult ODP in mice, and this enhancement was abolished by V1 infusion of nAChR antagonists or diazepam ( Morishita et al., 2010 ). Treatment with the antidepressant drug fluoxetine, a serotonin/noradrenaline reuptake inhibitor, also increased adult ODP in rats, an effect that was also abolished by infusion of diazepam into V1 ( Maya Vetencourt et al., 2008 ). Collectively, these two studies demonstrate that reduced neuromodulatory drive may hinder adult ODP, possibly by perturbing inhibitory function. However, since neuromodulators have widespread effects on network activity, they may directly modulate a number of circuits.
The maturation of structural factors that restrict the remodeling of circuits may also promote the closure of the critical period. Consistent with inhibited circuit remodeling in adults, a recent study showed that a prior episode of MD during the critical period facilitates subsequent ODP in the adult, possibly by establishing connections during the initial MD that persisted and could be made more potent when called on again in adulthood ( Hofer et al., 2006 , 2009 ). The maturation of perineuronal nets (PNNs) of the extracellular matrix (ECM) in adulthood ( Celio et al., 1998 ) has been proposed to inhibit remodeling of connections, and disrupting them enhanced adult ODP ( Carulli et al., 2010 ). Other more widely distributed structural factors that inhibit anatomical remodeling, such as the maturation of myelin, may also contribute to the diminished plasticity in adulthood. CNS myelination increases throughout the layers of V1 as the critical period closes ( McGee et al., 2005 ). Mice mutant for the Nogo-66 receptor (NgR), the Nogo/MAG/OMgp receptor PirB, or the NgR ligands, Nogo-A/B, all disrupted myelination and had enhanced adult ODP ( Atwal et al., 2008 ; McGee et al., 2005 ; Syken et al., 2006 ). Despite these encouraging experiments, it is still unknown whether the maturation of PNNs and myelination simply correlate with the closing of critical period ODP or are responsible for its closure.
Although multiple factors seem to be involved in closing the critical period and in inhibiting adult ODP, it is still unclear whether they are behaving in concert or independently. Heterochronic transplantation of inhibitory neuron precursors isolated from the medial ganglionic eminences of 12- to 16-day-old embryos into postnatal mice produced a second period of plasticity 33–35 days after transplantation, an age that matches that of host inhibitory neurons at the normal peak of the critical period ( Southwell et al., 2010 ). At the time of this second period of plasticity, the transplanted precursors had developed into a diverse set of inhibitory neurons with mature morphologies that made and received about three times as many connections with host excitatory neurons as host inhibitory neurons, and the transplant connections were about one-third the strength. The widespread connections of transplanted inhibitory neurons may have created a second critical period by destabilizing the mature network of host connections, by adding a new pattern of inhibition, or by providing a molecular signal that promotes plasticity. Further studies using heterochronic transplantations have the potential to determine the most pertinent factors involved in enhancing adult ODP.
Another feature of declining V1 plasticity in adulthood is the slow and incomplete recovery following long-term MD induced during the critical period. Reverse suture, or binocular experience alone are not potent enough to recover visual acuity ( Iny et al., 2006 ). A number of manipulations used to enhance adult ODP discussed above also allow recovery of acuity after long-term MD.
Is Enhancing Adult ODP the Same as Reopening the Critical Period?
To fully understand why and how the brain becomes less plastic with age, we must understand the differences between adult and critical period ODP. Studies that enhance adult ODP may simply be increasing the levels of adult plasticity rather than opening a second critical period like that observed in juvenile animals. Critical period plasticity is open for a limited duration of time and differs from adult ODP in a number of respects discussed above. Full reopening of the critical period probably involves reactivating an entire array of early plasticity mechanisms that are normally active during the critical period and inactivating many factors that impede adult ODP.
For a second period of ODP to resemble the normal critical period, three conditions should be met. First, manipulations that enhance adult ODP should cause the same changes in eye-specific responses as observed during the critical period ( Figure 5 ). Second, the time course of the plastic period should be like that of the normal critical period; it must be of limited duration. Third, the molecular mechanisms involved should play a similar role as in the normal critical period. None of the studies above have addressed all three issues together.
What Is the Purpose of Adult Plasticity?
To understand the decline in plasticity in adult V1, it may be helpful to first understand what purpose it serves. In zebra finches, active auditory feedback in adulthood is required for maintaining and continuously calibrating the song that was learned as juveniles ( Brainard and Doupe, 2000 ). If these principles apply to the mouse V1, circuits involved in persistent adult plasticity may be important for continuous fine tuning of visual responses, and perhaps for maintaining the binocular matching of receptive fields. Experiments to measure whether receptive fields remain stable and matched in the two eyes when adult plasticity is blocked or enhanced may illuminate the role of normal adult plasticity.
Identifying Neural Circuit Substrates of ODP
The classical studies by Hubel and Wiesel on ODP revealed that different elements of the neural circuit in V1 have different critical periods, suggesting that circuits have distinct roles ( LeVay et al., 1980 ). Presently, very little is known about which intracortical circuits are reconfigured in ODP. It also remains unclear whether the same circuits that are required for the opening of the critical period are those altered in its expression. Similarly, we do not know whether different, similar, or only a subset of the circuits involved in critical period ODP are reconfigured in adult ODP. Observing the anatomical and physiological changes in specific subsets of neurons, preferably longitudinally in the same mouse, promises to provide insight into the developmentally regulated mechanisms of ODP.
Below we discuss pharmacological and genetic manipulations that point to PV cells as regulators of the opening of the critical period. We then discuss recent studies that have used genetic labeling methods and longitudinal two-photon imaging to measure physiological and structural changes in specific circuits during ODP induced by MD in vivo. Future studies will require thorough characterization of specific neuronal populations, including concurrent longitudinal measures of physiology and structure during ODP with or without genetic and pharmacological manipulations.
PV Cells and the Opening of Critical Period ODP
Among the heterogeneous population of inhibitory interneurons, fast-spiking PV basket cells have been most clearly implicated in opening the critical period of ODP. PV cells receive direct thalamic input ( Cruikshank et al., 2007 ), synapse predominantly onto somata and proximal dendrites that use GABA A receptor α1 subunits ( Klausberger et al., 2002 ), and generate gamma-frequency (30–80 Hz) rhythmicity, which is important for sensory processing and learning ( Sohal et al., 2009 ). PV cell maturation is experience dependent and correlates with the opening of critical period ODP ( Chattopadhyaya et al., 2004 ). Manipulations that delay the opening of the critical period also delay the development of perisomatic synapses, and manipulations that promote earlier maturation of perisomatic synapses induce a precocious critical period ( Huang et al., 2007 ). Knockout of Otx2 prevents the maturation of PV cells and prevents the opening of the critical period ( Sugiyama et al., 2008 ). Consistent with the role of PV cells, activation of GABA A receptor α1 subunits can open a precocious critical period ( Fagiolini et al., 2004 ), although this class of receptors may also involve other inhibitory interneurons ( Klausberger et al., 2002 ).
An open question that remains is how the maturation of PV cells opens the critical period. PV cells could influence neuronal synchrony, influence spike timing-dependent plasticity, or instruct plasticity specifically in deprived-eye circuits. To test these effects would require temporally precise manipulation of activity in PV cells. The role of other cell types should also be investigated with the use of mice expressing the Cre recombinase in specific interneuron subsets ( Taniguchi et al., 2011 ).
Changes in the Responses of Excitatory and Inhibitory Neurons during ODP
As an initial parcelation of the neural circuits involved in plasticity, three studies have directly compared the ocular dominance of excitatory and inhibitory neurons following MD in critical period or adult mice.
Gandhi et al. (2008) used knockin mice in which GFP expression under the control of the GAD67 promoter labels most inhibitory neurons ( Tamamaki et al., 2003 ). Calcium imaging of neuronal responses in vivo demonstrated that both excitatory and inhibitory neurons undergo an ocular dominance shift toward the nondeprived eye after 4–6 days of MD; however, brief 2 day MD resulted in an ocular dominance shift in excitatory, but not inhibitory, neurons ( Gandhi et al., 2008 ). Thus, plasticity was faster in excitatory neurons.
Yazaki-Sugiyama et al. (2009) employed sharp microelectrodes to measure visual responses in excitatory cells and inhibitory fast-spiking cells in vivo. Excitatory and inhibitory neurons exhibited a similar ocular dominance shift toward the nondeprived eye after prolonged MD; however, brief 3 day MD shifted the responses of excitatory neurons toward the open eye but shifted inhibitory neurons in the opposite direction, toward the deprived eye ( Yazaki-Sugiyama et al., 2009 ). Thus, the two classes of neurons follow a different course of plasticity.
Kameyama et al. (2010) used a transgenic mouse line in which expression of a GFP variant (Venus) under the control of the vesicular GABA transporter (VGAT) promoter labels most inhibitory neurons. In contrast to the first two studies, brief 2 day MD resulted in a similar ocular dominance shift toward the nondeprived eye in excitatory and inhibitory neurons. Yet, excitatory and inhibitory neurons were still distinct in that excitatory neurons had both a decrease in deprived-eye and an increase in nondeprived-eye responses, while inhibitory neurons had only the increase in nondeprived-eye responses.
All three studies agree that MD during the critical period ultimately shifts the responses of both excitatory and inhibitory neurons toward the open eye, while brief MD produces a stronger effect on excitatory neurons. Hebbian models of the V1 circuit that incorporate the smaller ocular dominance shift of inhibitory neurons after brief MD provide a potential explanation of the requirement for a threshold level of inhibition for ODP ( Gandhi et al., 2008 ; Yazaki-Sugiyama et al., 2009 ). It is not yet clear what differences among mouse strains, inhibitory cell types, or techniques account for the inconsistency in inhibitory neuron responses between the three studies.
Measuring Layer-Specific Structural Changes during ODP
In monkeys and cats, transneuronal labeling revealed a shrinkage of deprived-eye and complementary expansion of open-eye thalamocortical projections ( Hubel et al., 1977 ). However, thalamocortical axon rearrangement is much too slow to explain the rapid shift of ocular dominance during the critical period ( Antonini and Stryker, 1993b ). Indeed in cats, responses of neurons in layer 4 have not begun to shift at 1–2 days MD when ocular dominance changes in layers 2–3 are nearly saturating ( Trachtenberg et al., 2000 ). This slower shift of ocular dominance in layer 4 parallels thalamocortical anatomical changes ( Antonini and Stryker, 1993b ). In contrast, anatomical changes in the upper layers of cortex are much more rapid: strabismus dramatically reduced horizontal connectivity between columns representing the two eyes in less than 2 days ( Trachtenberg and Stryker, 2001 ). Similarly, 4 days of MD had no effect on spine density in layer 4 spiny stellate neurons ( Lund et al., 1991 ). Interestingly, the difference in timing between ODP in layer 2/3 and layer 4 may not apply to the mouse ( Liu et al., 2008 ), in which thalamic inputs from the two eyes are intermingled in layer 4. In this situation, axon growth or retraction may not be required to find postsynaptic partners dominated by the other eye. This may also explain why rodents show more plasticity in adult life than do animals with a columnar cortical organization of V1 ( Lehmann and Löwel, 2008 ).
Measuring Structural Changes Longitudinally in Excitatory and Inhibitory Neurons during ODP
Structural and functional measurements can now delineate the inputs that give rise to specific response properties of different cell types in V1 ( Reid, 2012 ). Two-photon laser scanning imaging in mice also allows one to follow structural changes longitudinally during ODP. In critical period transgenic mice expressing GFP in a subset of layer 5 cells (thy1-GFP line M) ( Feng et al., 2000 ), the motility of spines in layers 2, 3, and 5, but not 4 was elevated by 2 days of MD ( Oray et al., 2004 ), consistent with early extragranular changes that instruct later events in layer 4 ( Trachtenberg et al., 2000 ). Since this effect was observed only in the binocular zone of V1, it probably reflects a competitive mechanism related to ODP.
In adult thy1-GFP line M mice, MD caused the addition of dendritic spines on the apical tufts of layer 5 but not layer 2/3 pyramidal neurons ( Hofer et al., 2009 ). Imaging of layer 2/3 in adult mice revealed much greater baseline turnover of distal dendritic branch tips in inhibitory than in excitatory neurons and particularly high turnover in the top half of layer 2/3 ( Lee et al., 2008 , 2006 ). MD increased turnover and led to a transient decrease in inhibitory tone ( Chen et al., 2011 ). Consistent with the findings above, immunohistochemistry of VGAT revealed a loss of inhibitory synapses onto layer 5 apical dendrites, but not the neighboring dendrites of layer 2/3 pyramidal neurons ( Chen et al., 2011 ). MD and recovery in adult mice each produce a transient loss of Gephyrin-labeled inhibitory synapses on spine heads of excitatory neurons, but not on their dendritic shafts ( Chen et al., 2012 ; van Versendaal et al., 2012 ). The spine heads themselves showed little change, suggesting that excitatory connections were stable ( Chen et al., 2012 ).
Outstanding Questions about the Development and Plasticity of V1
Considering the events we discuss in the development of V1, there is a satisfying account of topographic map formation. The next major event, the formation of highly selective receptive fields, remains largely a mystery. We do not know the details of the neural circuitry that gives rise to selective responses, and we lack experimental confirmation of the mechanisms responsible for its formation. The emergence of binocular responses in V1 seems to require no explanation beyond the convergence of eye-specific thalamocortical inputs, but the matching of preferred orientation in the two eyes suggest that experience dependent plasticity sculpts these circuits during normal development. A great deal is known about the mechanisms responsible for changes in binocular responses following MD in the critical period, and about some of the accompanying changes in the neural circuit, but it is not clear which of these mechanisms is responsible for the normal process of binocular matching. Finally, it is not yet clear how adult plasticity differs mechanistically and functionally from that of the critical period ( Figure 7 ).
These questions can now be addressed using a number of new tools for tracking neural activity, structure, and biochemical signaling pathways in individual cells over the course of development and plasticity. Observations can be targeted to specific cells in V1 using many novel brain region-, cortical layer-, and neuronal subtype-specific Cre-transgenic mice ( Madisen et al., 2010 ) in combination with Cre-dependent structural or physiological markers ( Bernard et al., 2009 ; Luo et al., 2008 ). Activity can be measured in the targeted cells using chemical and protein-based fluorescent biosensors of intracellular calcium ( Hasan et al., 2004 ; Mank et al., 2008 ; Tian et al., 2009 ), vesicle release ( Li and Tsien, 2012 ), or voltage ( Miller et al., 2012 ). Structural rearrangements can be measured in targeted cells along with fluorescently tagged synaptic proteins, including those that are newly synthesized and those that may indicate the strength of synaptic connections ( Lin et al., 2008 ). The development of true transsynaptic tracers also makes it possible, at least in principle, to study the properties of the neurons that provide input to a cell of interest ( Wickersham et al., 2007 ). Biochemical signaling mechanisms can be measured in these neurons using a number of new fluorescent probes ( Tsien, 2010 ). Changes in any of these properties in subsets of neurons at particular points in development or plasticity would point to a potential role of that element in the process.
New tools also exist for perturbing activity and biochemical signaling in particular cells with temporal precision. Transgenic mice expressing light sensitive ion channels and ion pumps ( Madisen et al., 2012 ), such as channelrhodopsin, halorhodopsin, and archaerhodopsin, make it possible to control the activity of specific neurons in either direction in living organisms by light pulses ( Chow et al., 2012 ; Fenno et al., 2011 ). Similarly, mutant G protein-coupled receptors that are exclusively activated by designer drugs (DREADDs) allow control of activity in either direction and for many hours ( Rogan and Roth, 2011 ). The signaling of specific kinases and other biochemical signals can also be manipulated transiently at a specific time in targeted cells in either direction ( Dar and Shokat, 2011 ; Fenno et al., 2011 ). These perturbations should allow true causal experiments to test the potential roles of different elements of the V1 circuit in development and plasticity.
The combination of these new tools allow questions about the relationship between functional and structural changes to be addressed. For example, the question posed in Figure 6 : Do responses during ODP change because the circuit has been rewired, or does the circuit get rewired because responses have changed? Knowing the relationship between neural activity, structure, and biochemical signals in particular cells and perturbing these at specific times is essential to answering this question.
Concluding Remarks
The research program initiated 50 years ago by Hubel and Wiesel has been tremendously fruitful. Much of our understanding of the organization, development, and plasticity of the neocortex comes from studies of V1 and related areas. The issues illuminated include the distinction between innate and experience-dependent mechanisms of development, the roles of molecular signals and patterned activity in organizing connections in development, critical periods in development, and many aspects of plasticity and recovery of function. Studies of the visual cortex have delineated simple rules that make sense of much of the staggering complexity of cortical circuits.
Nevertheless, our current understanding is far from adequate. Our mental models lump together many different kinds of neurons that surely must behave differently. We talk about the supragranular and subgranular cortex as if they were units, when in reality each is composed of many distinct cells.
Yet the best days for the study of the visual cortex lie ahead. New optical, molecular, and electrical methods for observing and perturbing specific, identified neurons open a new world of understanding. It is now becoming possible to study the cells and circuits of the visual cortex at the level at which they really operate, that of the connections among them and the patterns of activity that they convey. Plasticity can now or soon be followed longitudinally in identified cells of identified function in vivo. It should soon be possible to predict what will happen to each element of the visual cortical circuit that we observe when the animal has a particular experience to induce plasticity. One can hardly wait to see what the next 50 years will bring.
ACKNOWLEDGMENTS
Preparation of this manuscript was supported by NIH Grants R01-EY02874 to M.P.S. and F32-EY19613 to J.S.E. We are grateful to Cristopher Niell, Sunil Gandhi, Kathleen Cho, Yu Fu, Dan Darcy, and Jason Chung for critical readings of the manuscript.
- Akerman CJ, Smyth D, Thompson ID. Visual experience before eye-opening and the development of the retinogeniculate pathway. Neuron. 2002;36:869–879. doi: 10.1016/s0896-6273(02)01010-3. [ DOI ] [ PubMed ] [ Google Scholar ]
- Antonini A, Stryker MP. Development of individual geniculocortical arbors in cat striate cortex and effects of binocular impulse blockade. J. Neurosci. 1993a;13:3549–3573. doi: 10.1523/JNEUROSCI.13-08-03549.1993. [ DOI ] [ PMC free article ] [ PubMed ] [ Google Scholar ]
- Antonini A, Stryker MP. Rapid remodeling of axonal arbors in the visual cortex. Science. 1993b;260:1819–1821. doi: 10.1126/science.8511592. [ DOI ] [ PubMed ] [ Google Scholar ]
- Antonini A, Fagiolini M, Stryker MP. Anatomical correlates of functional plasticity in mouse visual cortex. J. Neurosci. 1999;19:4388–4406. doi: 10.1523/JNEUROSCI.19-11-04388.1999. [ DOI ] [ PMC free article ] [ PubMed ] [ Google Scholar ]
- Asada H, Kawamura Y, Maruyama K, Kume H, Ding RG, Kanbara N, Kuzume H, Sanbo M, Yagi T, Obata K. Cleft palate and decreased brain gamma-aminobutyric acid in mice lacking the 67-kDa isoform of glutamic acid decarboxylase. Proc. Natl. Acad. Sci. USA. 1997;94:6496–6499. doi: 10.1073/pnas.94.12.6496. [ DOI ] [ PMC free article ] [ PubMed ] [ Google Scholar ]
- Atwal JK, Pinkston-Gosse J, Syken J, Stawicki S, Wu Y, Shatz C, Tessier-Lavigne M. PirB is a functional receptor for myelin inhibitors of axonal regeneration. Science. 2008;322:967–970. doi: 10.1126/science.1161151. [ DOI ] [ PubMed ] [ Google Scholar ]
- Bavelier D, Levi DM, Li RW, Dan Y, Hensch TK. Removing brakes on adult brain plasticity: from molecular to behavioral interventions. J. Neurosci. 2010;30:14964–14971. doi: 10.1523/JNEUROSCI.4812-10.2010. [ DOI ] [ PMC free article ] [ PubMed ] [ Google Scholar ]
- Bear MF, Singer W. Modulation of visual cortical plasticity by acetylcholine and noradrenaline. Nature. 1986;320:172–176. doi: 10.1038/320172a0. [ DOI ] [ PubMed ] [ Google Scholar ]
- Bear MF, Kleinschmidt A, Gu QA, Singer W. Disruption of experience-dependent synaptic modifications in striate cortex by infusion of an NMDA receptor antagonist. J. Neurosci. 1990;10:909–925. doi: 10.1523/JNEUROSCI.10-03-00909.1990. [ DOI ] [ PMC free article ] [ PubMed ] [ Google Scholar ]
- Berman N, Daw NW. Comparison of the critical periods for monocular and directional deprivation in cats. J. Physiol. 1977;265:249–259. doi: 10.1113/jphysiol.1977.sp011715. [ DOI ] [ PMC free article ] [ PubMed ] [ Google Scholar ]
- Bernard A, Sorensen SA, Lein ES. Shifting the paradigm: new approaches for characterizing and classifying neurons. Curr. Opin. Neurobiol. 2009;19:530–536. doi: 10.1016/j.conb.2009.09.010. [ DOI ] [ PubMed ] [ Google Scholar ]
- Blakemore C, Mitchell DE. Environmental modification of the visual cortex and the neural basis of learning and memory. Nature. 1973;241:467–468. doi: 10.1038/241467a0. [ DOI ] [ PubMed ] [ Google Scholar ]
- Bonhoeffer T. Neurotrophins and activity-dependent development of the neocortex. Curr. Opin. Neurobiol. 1996;6:119–126. doi: 10.1016/s0959-4388(96)80017-1. [ DOI ] [ PubMed ] [ Google Scholar ]
- Bonhoeffer T, Grinvald A. Optical imaging based on intrinsic signals. In: Toga AW, Mazziotta JC, editors. Brain mapping: The Methods. New York: Academic; 1996. pp. 55–97. [ Google Scholar ]
- Brainard MS, Doupe AJ. Interruption of a basal ganglia-fore-brain circuit prevents plasticity of learned vocalizations. Nature. 2000;404:762–766. doi: 10.1038/35008083. [ DOI ] [ PubMed ] [ Google Scholar ]
- Brainard MS, Doupe AJ. What songbirds teach us about learning. Nature. 2002;417:351–358. doi: 10.1038/417351a. [ DOI ] [ PubMed ] [ Google Scholar ]
- Cang J, Kaneko M, Yamada J, Woods G, Stryker MP, Feldheim DA. Ephrin-as guide the formation of functional maps in the visual cortex. Neuron. 2005a;48:577–589. doi: 10.1016/j.neuron.2005.10.026. [ DOI ] [ PMC free article ] [ PubMed ] [ Google Scholar ]
- Cang J, Rentería RC, Kaneko M, Liu X, Copenhagen DR, Stryker MP. Development of precise maps in visual cortex requires patterned spontaneous activity in the retina. Neuron. 2005b;48:797–809. doi: 10.1016/j.neuron.2005.09.015. [ DOI ] [ PMC free article ] [ PubMed ] [ Google Scholar ]
- Cang J, Niell CM, Liu X, Pfeiffenberger C, Feldheim DA, Stryker MP. Selective disruption of one Cartesian axis of cortical maps and receptive fields by deficiency in ephrin-As and structured activity. Neuron. 2008;57:511–523. doi: 10.1016/j.neuron.2007.12.025. [ DOI ] [ PMC free article ] [ PubMed ] [ Google Scholar ]
- Carulli D, Pizzorusso T, Kwok JC, Putignano E, Poli A, Forostyak S, Andrews MR, Deepa SS, Glant TT, Fawcett JW. Animals lacking link protein have attenuated perineuronal nets and persistent plasticity. Brain. 2010;133:2331–2347. doi: 10.1093/brain/awq145. [ DOI ] [ PubMed ] [ Google Scholar ]
- Celio MR, Spreafico R, De Biasi S, Vitellaro-Zuccarello L. Perineuronal nets: past and present. Trends Neurosci. 1998;21:510–515. doi: 10.1016/s0166-2236(98)01298-3. [ DOI ] [ PubMed ] [ Google Scholar ]
- Chang EF, Merzenich MM. Environmental noise retards auditory cortical development. Science. 2003;300:498–502. doi: 10.1126/science.1082163. [ DOI ] [ PubMed ] [ Google Scholar ]
- Chapman B, Stryker MP. Development of orientation selectivity in ferret visual cortex and effects of deprivation. J. Neurosci. 1993;13:5251–5262. doi: 10.1523/JNEUROSCI.13-12-05251.1993. [ DOI ] [ PMC free article ] [ PubMed ] [ Google Scholar ]
- Chapman B, Stryker MP, Bonhoeffer T. Development of orientation preference maps in ferret primary visual cortex. J. Neurosci. 1996;16:6443–6453. doi: 10.1523/JNEUROSCI.16-20-06443.1996. [ DOI ] [ PMC free article ] [ PubMed ] [ Google Scholar ]
- Chattopadhyaya B, Di Cristo G, Higashiyama H, Knott GW, Kuhlman SJ, Welker E, Huang ZJ. Experience and activity-dependent maturation of perisomatic GABAergic innervation in primary visual cortex during a postnatal critical period. J. Neurosci. 2004;24:9598–9611. doi: 10.1523/JNEUROSCI.1851-04.2004. [ DOI ] [ PMC free article ] [ PubMed ] [ Google Scholar ]
- Chen X, Ye H, Kuruvilla R, Ramanan N, Scangos KW, Zhang C, Johnson NM, England PM, Shokat KM, Ginty DD. A chemical-genetic approach to studying neurotrophin signaling. Neuron. 2005;46:13–21. doi: 10.1016/j.neuron.2005.03.009. [ DOI ] [ PubMed ] [ Google Scholar ]
- Chen JL, Lin WC, Cha JW, So PT, Kubota Y, Nedivi E. Structural basis for the role of inhibition in facilitating adult brain plasticity. Nat. Neurosci. 2011;14:587–594. doi: 10.1038/nn.2799. [ DOI ] [ PMC free article ] [ PubMed ] [ Google Scholar ]
- Chen JL, Villa KL, Cha JW, So PT, Kubota Y, Nedivi E. Clustered dynamics of inhibitory synapses and dendritic spines in the adult neocortex. Neuron. 2012;74:361–373. doi: 10.1016/j.neuron.2012.02.030. [ DOI ] [ PMC free article ] [ PubMed ] [ Google Scholar ]
- Chiu C, Weliky M. Spontaneous activity in developing ferret visual cortex in vivo. J. Neurosci. 2001;21:8906–8914. doi: 10.1523/JNEUROSCI.21-22-08906.2001. [ DOI ] [ PMC free article ] [ PubMed ] [ Google Scholar ]
- Cho KK, Khibnik L, Philpot BD, Bear MF. The ratio of NR2A/B NMDA receptor subunits determines the qualities of ocular dominance plasticity in visual cortex. Proc. Natl. Acad. Sci. USA. 2009;106:5377–5382. doi: 10.1073/pnas.0808104106. [ DOI ] [ PMC free article ] [ PubMed ] [ Google Scholar ]
- Chow BY, Han X, Boyden ES. Genetically encoded molecular tools for light-driven silencing of targeted neurons. Prog. Brain Res. 2012;196:49–61. doi: 10.1016/B978-0-444-59426-6.00003-3. [ DOI ] [ PMC free article ] [ PubMed ] [ Google Scholar ]
- Ciucci F, Putignano E, Baroncelli L, Landi S, Berardi N, Maffei L. Insulin-like growth factor 1 (IGF-1) mediates the effects of enriched environment (EE) on visual cortical development. PLoS ONE. 2007;2:e475. doi: 10.1371/journal.pone.0000475. [ DOI ] [ PMC free article ] [ PubMed ] [ Google Scholar ]
- Crair MC, Gillespie DC, Stryker MP. The role of visual experience in the development of columns in cat visual cortex. Science. 1998;279:566–570. doi: 10.1126/science.279.5350.566. [ DOI ] [ PMC free article ] [ PubMed ] [ Google Scholar ]
- Crair MC, Horton JC, Antonini A, Stryker MP. Emergence of ocular dominance columns in cat visual cortex by 2 weeks of age. J. Comp. Neurol. 2001;430:235–249. doi: 10.1002/1096-9861(20010205)430:2<235::aid-cne1028>3.0.co;2-p. [ DOI ] [ PMC free article ] [ PubMed ] [ Google Scholar ]
- Crowley JC, Katz LC. Early development of ocular dominance columns. Science. 2000;290:1321–1324. doi: 10.1126/science.290.5495.1321. [ DOI ] [ PubMed ] [ Google Scholar ]
- Cruikshank SJ, Lewis TJ, Connors BW. Synaptic basis for intense thalamocortical activation of feedforward inhibitory cells in neocortex. Nat. Neurosci. 2007;10:462–468. doi: 10.1038/nn1861. [ DOI ] [ PubMed ] [ Google Scholar ]
- Dar AC, Shokat KM. The evolution of protein kinase inhibitors from antagonists to agonists of cellular signaling. Annu. Rev. Biochem. 2011;80:769–795. doi: 10.1146/annurev-biochem-090308-173656. [ DOI ] [ PubMed ] [ Google Scholar ]
- Datwani A, McConnell MJ, Kanold PO, Micheva KD, Busse B, Shamloo M, Smith SJ, Shatz CJ. Classical MHCI molecules regulate retinogeniculate refinement and limit ocular dominance plasticity. Neuron. 2009;64:463–470. doi: 10.1016/j.neuron.2009.10.015. [ DOI ] [ PMC free article ] [ PubMed ] [ Google Scholar ]
- Daw NW. Visual Development. New York: Plenum Press; 1995. [ Google Scholar ]
- Daw NW, Fox K, Sato H, Czepita D. Critical period for monocular deprivation in the cat visual cortex. J. Neurophysiol. 1992;67:197–202. doi: 10.1152/jn.1992.67.1.197. [ DOI ] [ PubMed ] [ Google Scholar ]
- Daw NW, Stein PS, Fox K. The role of NMDA receptors in information processing. Annu. Rev. Neurosci. 1993;16:207–222. doi: 10.1146/annurev.ne.16.030193.001231. [ DOI ] [ PubMed ] [ Google Scholar ]
- Des Rosiers MH, Sakurada O, Jehle J, Shinohara M, Kennedy C, Sokoloff L. Functional plasticity in the immature striate cortex of the monkey shown by the [14C]deoxyglucose method. Science. 1978;200:447–449. doi: 10.1126/science.417397. [ DOI ] [ PubMed ] [ Google Scholar ]
- Di Cristo G, Berardi N, Cancedda L, Pizzorusso T, Putignano E, Ratto GM, Maffei L. Requirement of ERK activation for visual cortical plasticity. Science. 2001;292:2337–2340. doi: 10.1126/science.1059075. [ DOI ] [ PubMed ] [ Google Scholar ]
- Di Cristo G, Chattopadhyaya B, Kuhlman SJ, Fu Y, Bélanger MC, Wu CZ, Rutishauser U, Maffei L, Huang ZJ. Activity-dependent PSA expression regulates inhibitory maturation and onset of critical period plasticity. Nat. Neurosci. 2007;10:1569–1577. doi: 10.1038/nn2008. [ DOI ] [ PubMed ] [ Google Scholar ]
- Domenici L, Parisi V, Maffei L. Exogenous supply of nerve growth factor prevents the effects of strabismus in the rat. Neuroscience. 1992;51:19–24. doi: 10.1016/0306-4522(92)90466-f. [ DOI ] [ PubMed ] [ Google Scholar ]
- Dräger UC. Receptive fields of single cells and topography in mouse visual cortex. J. Comp. Neurol. 1975;160:269–290. doi: 10.1002/cne.901600302. [ DOI ] [ PubMed ] [ Google Scholar ]
- Dyck RH, Cynader MS. An interdigitated columnar mosaic of cytochrome oxidase, zinc, and neurotransmitter-related molecules in cat and monkey visual cortex. Proc. Natl. Acad. Sci. USA. 1993;90:9066–9069. doi: 10.1073/pnas.90.19.9066. [ DOI ] [ PMC free article ] [ PubMed ] [ Google Scholar ]
- Fagiolini M, Hensch TK. Inhibitory threshold for critical-period activation in primary visual cortex. Nature. 2000;404:183–186. doi: 10.1038/35004582. [ DOI ] [ PubMed ] [ Google Scholar ]
- Fagiolini M, Pizzorusso T, Berardi N, Domenici L, Maffei L. Functional postnatal development of the rat primary visual cortex and the role of visual experience: dark rearing and monocular deprivation. Vision Res. 1994;34:709–720. doi: 10.1016/0042-6989(94)90210-0. [ DOI ] [ PubMed ] [ Google Scholar ]
- Fagiolini M, Fritschy JM, Löw K, Möhler H, Rudolph U, Hensch TK. Specific GABAA circuits for visual cortical plasticity. Science. 2004;303:1681–1683. doi: 10.1126/science.1091032. [ DOI ] [ PubMed ] [ Google Scholar ]
- Feldheim DA, O’Leary DD. Visual map development: bidirectional signaling, bifunctional guidance molecules, and competition. Cold Spring Harb. Perspect. Biol. 2010;2:a001768. doi: 10.1101/cshperspect.a001768. [ DOI ] [ PMC free article ] [ PubMed ] [ Google Scholar ]
- Feng G, Mellor RH, Bernstein M, Keller-Peck C, Nguyen QT, Wallace M, Nerbonne JM, Lichtman JW, Sanes JR. Imaging neuronal subsets in transgenic mice expressing multiple spectral variants of GFP. Neuron. 2000;28:41–51. doi: 10.1016/s0896-6273(00)00084-2. [ DOI ] [ PubMed ] [ Google Scholar ]
- Fenno L, Yizhar O, Deisseroth K. The development and application of optogenetics. Annu. Rev. Neurosci. 2011;34:389–412. doi: 10.1146/annurev-neuro-061010-113817. [ DOI ] [ PMC free article ] [ PubMed ] [ Google Scholar ]
- Fischer QS, Beaver CJ, Yang Y, Rao Y, Jakobsdottir KB, Storm DR, McKnight GS, Daw NW. Requirement for the RIIbeta isoform of PKA, but not calcium-stimulated adenylyl cyclase, in visual cortical plasticity. J. Neurosci. 2004;24:9049–9058. doi: 10.1523/JNEUROSCI.2409-04.2004. [ DOI ] [ PMC free article ] [ PubMed ] [ Google Scholar ]
- Fox K. A critical period for experience-dependent synaptic plasticity in rat barrel cortex. J. Neurosci. 1992;12:1826–1838. doi: 10.1523/JNEUROSCI.12-05-01826.1992. [ DOI ] [ PMC free article ] [ PubMed ] [ Google Scholar ]
- Frenkel MY, Sawtell NB, Diogo AC, Yoon B, Neve RL, Bear MF. Instructive effect of visual experience in mouse visual cortex. Neuron. 2006;51:339–349. doi: 10.1016/j.neuron.2006.06.026. [ DOI ] [ PubMed ] [ Google Scholar ]
- Gandhi SP, Cang J, Stryker MP. An eye-opening experience. Nat. Neurosci. 2005;8:9–10. doi: 10.1038/nn0105-9. [ DOI ] [ PMC free article ] [ PubMed ] [ Google Scholar ]
- Gandhi SP, Yanagawa Y, Stryker MP. Delayed plasticity of inhibitory neurons in developing visual cortex. Proc. Natl. Acad. Sci. USA. 2008;105:16797–16802. doi: 10.1073/pnas.0806159105. [ DOI ] [ PMC free article ] [ PubMed ] [ Google Scholar ]
- Gianfranceschi L, Siciliano R, Walls J, Morales B, Kirkwood A, Huang ZJ, Tonegawa S, Maffei L. Visual cortex is rescued from the effects of dark rearing by overexpression of BDNF. Proc. Natl. Acad. Sci. USA. 2003;100:12486–12491. doi: 10.1073/pnas.1934836100. [ DOI ] [ PMC free article ] [ PubMed ] [ Google Scholar ]
- Gilbert CD, Li W. Adult visual cortical plasticity. Neuron. 2012;75:250–264. doi: 10.1016/j.neuron.2012.06.030. this issue. [ DOI ] [ PMC free article ] [ PubMed ] [ Google Scholar ]
- Gödecke I, Bonhoeffer T. Development of identical orientation maps for two eyes without common visual experience. Nature. 1996;379:251–254. doi: 10.1038/379251a0. [ DOI ] [ PubMed ] [ Google Scholar ]
- Gordon JA, Stryker MP. Experience-dependent plasticity of binocular responses in the primary visual cortex of the mouse. J. Neurosci. 1996;16:3274–3286. doi: 10.1523/JNEUROSCI.16-10-03274.1996. [ DOI ] [ PMC free article ] [ PubMed ] [ Google Scholar ]
- Guire ES, Lickey ME, Gordon B. Critical period for the monocular deprivation effect in rats: assessment with sweep visually evoked potentials. J. Neurophysiol. 1999;81:121–128. doi: 10.1152/jn.1999.81.1.121. [ DOI ] [ PubMed ] [ Google Scholar ]
- Hanover JL, Huang ZJ, Tonegawa S, Stryker MP. Brain-derived neurotrophic factor overexpression induces precocious critical period in mouse visual cortex. J. Neurosci. 1999;19:RC40. doi: 10.1523/JNEUROSCI.19-22-j0003.1999. [ DOI ] [ PMC free article ] [ PubMed ] [ Google Scholar ]
- Harauzov A, Spolidoro M, DiCristo G, De Pasquale R, Cancedda L, Pizzorusso T, Viegi A, Berardi N, Maffei L. Reducing intracortical inhibition in the adult visual cortex promotes ocular dominance plasticity. J. Neurosci. 2010;30:361–371. doi: 10.1523/JNEUROSCI.2233-09.2010. [ DOI ] [ PMC free article ] [ PubMed ] [ Google Scholar ]
- Hasan MT, Friedrich RW, Euler T, Larkum ME, Giese G, Both M, Duebel J, Waters J, Bujard H, Griesbeck O, et al. Functional fluorescent Ca2+ indicator proteins in transgenic mice under TET control. PLoS Biol. 2004;2:e163. doi: 10.1371/journal.pbio.0020163. [ DOI ] [ PMC free article ] [ PubMed ] [ Google Scholar ]
- Hensch TK, Fagiolini M, Mataga N, Stryker MP, Baekkeskov S, Kash SF. Local GABA circuit control of experience-dependent plasticity in developing visual cortex. Science. 1998;282:1504–1508. doi: 10.1126/science.282.5393.1504. [ DOI ] [ PMC free article ] [ PubMed ] [ Google Scholar ]
- Hofer SB, Mrsic-Flogel TD, Bonhoeffer T, Hübener M. Prior experience enhances plasticity in adult visual cortex. Nat. Neurosci. 2006;9:127–132. doi: 10.1038/nn1610. [ DOI ] [ PubMed ] [ Google Scholar ]
- Hofer SB, Mrsic-Flogel TD, Bonhoeffer T, Hübener M. Experience leaves a lasting structural trace in cortical circuits. Nature. 2009;457:313–317. doi: 10.1038/nature07487. [ DOI ] [ PMC free article ] [ PubMed ] [ Google Scholar ]
- Horton JC, Hocking DR. An adult-like pattern of ocular dominance columns in striate cortex of newborn monkeys prior to visual experience. J. Neurosci. 1996;16:1791–1807. doi: 10.1523/JNEUROSCI.16-05-01791.1996. [ DOI ] [ PMC free article ] [ PubMed ] [ Google Scholar ]
- Horton JC, Hocking DR. Timing of the critical period for plasticity of ocular dominance columns in macaque striate cortex. J. Neurosci. 1997;17:3684–3709. doi: 10.1523/JNEUROSCI.17-10-03684.1997. [ DOI ] [ PMC free article ] [ PubMed ] [ Google Scholar ]
- Hoyt CS. Amblyopia: a neuro-ophthalmic view. J. Neuroophthalmol. 2005;25:227–231. doi: 10.1097/01.wno.0000177304.67715.ba. [ DOI ] [ PubMed ] [ Google Scholar ]
- Huang ZJ, Kirkwood A, Pizzorusso T, Porciatti V, Morales B, Bear MF, Maffei L, Tonegawa S. BDNF regulates the maturation of inhibition and the critical period of plasticity in mouse visual cortex. Cell. 1999;98:739–755. doi: 10.1016/s0092-8674(00)81509-3. [ DOI ] [ PubMed ] [ Google Scholar ]
- Huang ZJ, Di Cristo G, Ango F. Development of GABA innervation in the cerebral and cerebellar cortices. Nat. Rev. Neurosci. 2007;8:673–686. doi: 10.1038/nrn2188. [ DOI ] [ PubMed ] [ Google Scholar ]
- Hubel DH, Wiesel TN. Receptive fields, binocular interaction and functional architecture in the cat’s visual cortex. J. Physiol. 1962;160:106–154. doi: 10.1113/jphysiol.1962.sp006837. [ DOI ] [ PMC free article ] [ PubMed ] [ Google Scholar ]
- Hubel DH, Wiesel TN. Receptive Fields of Cells in Striate Cortex of Very Young, Visually Inexperienced Kittens. J. Neurophysiol. 1963;26:994–1002. doi: 10.1152/jn.1963.26.6.994. [ DOI ] [ PubMed ] [ Google Scholar ]
- Hubel DH, Wiesel TN. Binocular interaction in striate cortex of kittens reared with artificial squint. J. Neurophysiol. 1965;28:1041–1059. doi: 10.1152/jn.1965.28.6.1041. [ DOI ] [ PubMed ] [ Google Scholar ]
- Hubel DH, Wiesel TN. The period of susceptibility to the physiological effects of unilateral eye closure in kittens. J. Physiol. 1970;206:419–436. doi: 10.1113/jphysiol.1970.sp009022. [ DOI ] [ PMC free article ] [ PubMed ] [ Google Scholar ]
- Hubel DH, Wiesel TN, LeVay S. Functional architecture of area 17 in normal and monocularly deprived macaque monkeys. Cold Spring Harb. Symp. Quant. Biol. 1976;40:581–589. doi: 10.1101/sqb.1976.040.01.054. [ DOI ] [ PubMed ] [ Google Scholar ]
- Hubel DH, Wiesel TN, LeVay S. Plasticity of ocular dominance columns in monkey striate cortex. Philos. Trans. R. Soc. Lond. B Biol. Sci. 1977;278:377–409. doi: 10.1098/rstb.1977.0050. [ DOI ] [ PubMed ] [ Google Scholar ]
- Iny K, Heynen AJ, Sklar E, Bear MF. Bidirectional modifications of visual acuity induced by monocular deprivation in juvenile and adult rats. J. Neurosci. 2006;26:7368–7374. doi: 10.1523/JNEUROSCI.0124-06.2006. [ DOI ] [ PMC free article ] [ PubMed ] [ Google Scholar ]
- Issa NP, Trachtenberg JT, Chapman B, Zahs KR, Stryker MP. The critical period for ocular dominance plasticity in the Ferret’s visual cortex. J. Neurosci. 1999;19:6965–6978. doi: 10.1523/JNEUROSCI.19-16-06965.1999. [ DOI ] [ PMC free article ] [ PubMed ] [ Google Scholar ]
- Iwai Y, Fagiolini M, Obata K, Hensch TK. Rapid critical period induction by tonic inhibition in visual cortex. J. Neurosci. 2003;23:6695–6702. doi: 10.1523/JNEUROSCI.23-17-06695.2003. [ DOI ] [ PMC free article ] [ PubMed ] [ Google Scholar ]
- Johansen JP, Cain CK, Ostroff LE, LeDoux JE. Molecular mechanisms of fear learning and memory. Cell. 2011;147:509–524. doi: 10.1016/j.cell.2011.10.009. [ DOI ] [ PMC free article ] [ PubMed ] [ Google Scholar ]
- Kameyama K, Sohya K, Ebina T, Fukuda A, Yanagawa Y, Tsumoto T. Difference in binocularity and ocular dominance plasticity between GABAergic and excitatory cortical neurons. J. Neurosci. 2010;30:1551–1559. doi: 10.1523/JNEUROSCI.5025-09.2010. [ DOI ] [ PMC free article ] [ PubMed ] [ Google Scholar ]
- Kaneko M, Hanover JL, England PM, Stryker MP. TrkB kinase is required for recovery, but not loss, of cortical responses following monocular deprivation. Nat. Neurosci. 2008a;11:497–504. doi: 10.1038/nn2068. [ DOI ] [ PMC free article ] [ PubMed ] [ Google Scholar ]
- Kaneko M, Stellwagen D, Malenka RC, Stryker MP. Tumor necrosis factor-alpha mediates one component of competitive, experience-dependent plasticity in developing visual cortex. Neuron. 2008b;58:673–680. doi: 10.1016/j.neuron.2008.04.023. [ DOI ] [ PMC free article ] [ PubMed ] [ Google Scholar ]
- Kaneko M, Cheetham CE, Lee YS, Silva AJ, Stryker MP, Fox K. Constitutively active H-ras accelerates multiple forms of plasticity in developing visual cortex. Proc. Natl. Acad. Sci. USA. 2010;107:19026–19031. doi: 10.1073/pnas.1013866107. [ DOI ] [ PMC free article ] [ PubMed ] [ Google Scholar ]
- Kaneko M, Xie Y, An JJ, Stryker MP, Xu B. Dendritic BDNF synthesis is required for late-phase spine maturation and recovery of cortical responses following sensory deprivation. J. Neurosci. 2012;32:4790–4802. doi: 10.1523/JNEUROSCI.4462-11.2012. [ DOI ] [ PMC free article ] [ PubMed ] [ Google Scholar ]
- Kanold PO, Luhmann HJ. The subplate and early cortical circuits. Annu. Rev. Neurosci. 2010;33:23–48. doi: 10.1146/annurev-neuro-060909-153244. [ DOI ] [ PubMed ] [ Google Scholar ]
- Kasthuri N, Lichtman JW. The role of neuronal identity in synaptic competition. Nature. 2003;424:426–430. doi: 10.1038/nature01836. [ DOI ] [ PubMed ] [ Google Scholar ]
- Kauderer BS, Kandel ER. Capture of a protein synthesis-dependent component of long-term depression. Proc. Natl. Acad. Sci. USA. 2000;97:13342–13347. doi: 10.1073/pnas.97.24.13342. [ DOI ] [ PMC free article ] [ PubMed ] [ Google Scholar ]
- Kawasaki H, Crowley JC, Livesey FJ, Katz LC. Molecular organization of the ferret visual thalamus. J. Neurosci. 2004;24:9962–9970. doi: 10.1523/JNEUROSCI.2165-04.2004. [ DOI ] [ PMC free article ] [ PubMed ] [ Google Scholar ]
- Klausberger T, Roberts JD, Somogyi P. Cell type- and input-specific differences in the number and subtypes of synaptic GABA(A) receptors in the hippocampus. J. Neurosci. 2002;22:2513–2521. doi: 10.1523/JNEUROSCI.22-07-02513.2002. [ DOI ] [ PMC free article ] [ PubMed ] [ Google Scholar ]
- Knudsen EI, Zheng W, DeBello WM. Traces of learning in the auditory localization pathway. Proc. Natl. Acad. Sci. USA. 2000;97:11815–11820. doi: 10.1073/pnas.97.22.11815. [ DOI ] [ PMC free article ] [ PubMed ] [ Google Scholar ]
- Ko H, Hofer SB, Pichler B, Buchanan KA, Sjöström PJ, Mrsic-Flogel TD. Functional specificity of local synaptic connections in neocortical networks. Nature. 2011;473:87–91. doi: 10.1038/nature09880. [ DOI ] [ PMC free article ] [ PubMed ] [ Google Scholar ]
- Kreile AK, Bonhoeffer T, Hübener M. Altered visual experience induces instructive changes of orientation preference in mouse visual cortex. J. Neurosci. 2011;31:13911–13920. doi: 10.1523/JNEUROSCI.2143-11.2011. [ DOI ] [ PMC free article ] [ PubMed ] [ Google Scholar ]
- Lee WC, Huang H, Feng G, Sanes JR, Brown EN, So PT, Nedivi E. Dynamic remodeling of dendritic arbors in GABAergic interneurons of adult visual cortex. PLoS Biol. 2006;4:e29. doi: 10.1371/journal.pbio.0040029. [ DOI ] [ PMC free article ] [ PubMed ] [ Google Scholar ]
- Lee WC, Chen JL, Huang H, Leslie JH, Amitai Y, So PT, Nedivi E. A dynamic zone defines interneuron remodeling in the adult neocortex. Proc. Natl. Acad. Sci. USA. 2008;105:19968–19973. doi: 10.1073/pnas.0810149105. [ DOI ] [ PMC free article ] [ PubMed ] [ Google Scholar ]
- Lehmann K, Löwel S. Age-dependent ocular dominance plasticity in adult mice. PLoS ONE. 2008;3:e3120. doi: 10.1371/journal.pone.0003120. [ DOI ] [ PMC free article ] [ PubMed ] [ Google Scholar ]
- Lenneberg EH. Biological Foundations of Language. New York: Wiley; 1967. [ Google Scholar ]
- LeVay S, Stryker MP, Shatz CJ. Ocular dominance columns and their development in layer IV of the cat’s visual cortex: a quantitative study. J. Comp. Neurol. 1978;179:223–244. doi: 10.1002/cne.901790113. [ DOI ] [ PubMed ] [ Google Scholar ]
- LeVay S, Wiesel TN, Hubel DH. The development of ocular dominance columns in normal and visually deprived monkeys. J. Comp. Neurol. 1980;191:1–51. doi: 10.1002/cne.901910102. [ DOI ] [ PubMed ] [ Google Scholar ]
- Li Y, Tsien RW. pHTomato, a red, genetically encoded indicator that enables multiplex interrogation of synaptic activity. Nat. Neurosci. 2012;15:1047–1053. doi: 10.1038/nn.3126. [ DOI ] [ PMC free article ] [ PubMed ] [ Google Scholar ]
- Li Z, Sheng M. Caspases in synaptic plasticity. Mol. Brain. 2012;5:15. doi: 10.1186/1756-6606-5-15. [ DOI ] [ PMC free article ] [ PubMed ] [ Google Scholar ]
- Li Y, Van Hooser SD, Mazurek M, White LE, Fitzpatrick D. Experience with moving visual stimuli drives the early development of cortical direction selectivity. Nature. 2008;456:952–956. doi: 10.1038/nature07417. [ DOI ] [ PMC free article ] [ PubMed ] [ Google Scholar ]
- Lin MZ, Glenn JS, Tsien RY. A drug-controllable tag for visualizing newly synthesized proteins in cells and whole animals. Proc. Natl. Acad. Sci. USA. 2008;105:7744–7749. doi: 10.1073/pnas.0803060105. [ DOI ] [ PMC free article ] [ PubMed ] [ Google Scholar ]
- Liu CH, Heynen AJ, Shuler MG, Bear MF. Cannabinoid receptor blockade reveals parallel plasticity mechanisms in different layers of mouse visual cortex. Neuron. 2008;58:340–345. doi: 10.1016/j.neuron.2008.02.020. [ DOI ] [ PubMed ] [ Google Scholar ]
- Lorenz KZ. The evolution of behavior. Sci. Am. 1958;199:67–74. doi: 10.1038/scientificamerican1258-67. [ DOI ] [ PubMed ] [ Google Scholar ]
- Lund JS, Holbach SM, Chung WW. Postnatal development of thalamic recipient neurons in the monkey striate cortex: II. Influence of afferent driving on spine acquisition and dendritic growth of layer 4C spiny stellate neurons. J. Comp. Neurol. 1991;309:129–140. doi: 10.1002/cne.903090109. [ DOI ] [ PubMed ] [ Google Scholar ]
- Luo L, Callaway EM, Svoboda K. Genetic dissection of neural circuits. Neuron. 2008;57:634–660. doi: 10.1016/j.neuron.2008.01.002. [ DOI ] [ PMC free article ] [ PubMed ] [ Google Scholar ]
- Madisen L, Zwingman TA, Sunkin SM, Oh SW, Zariwala HA, Gu H, Ng LL, Palmiter RD, Hawrylycz MJ, Jones AR, et al. A robust and high-throughput Cre reporting and characterization system for the whole mouse brain. Nat. Neurosci. 2010;13:133–140. doi: 10.1038/nn.2467. [ DOI ] [ PMC free article ] [ PubMed ] [ Google Scholar ]
- Madisen L, Mao T, Koch H, Zhuo JM, Berenyi A, Fujisawa S, Hsu YW, Garcia AJ, 3rd, Gu X, Zanella S, et al. A toolbox of Cre-dependent optogenetic transgenic mice for light-induced activation and silencing. Nat. Neurosci. 2012;15:793–802. doi: 10.1038/nn.3078. [ DOI ] [ PMC free article ] [ PubMed ] [ Google Scholar ]
- Mank M, Santos AF, Direnberger S, Mrsic-Flogel TD, Hofer SB, Stein V, Hendel T, Reiff DF, Levelt C, Borst A, et al. A genetically encoded calcium indicator for chronic in vivo two-photon imaging. Nat. Methods. 2008;5:805–811. doi: 10.1038/nmeth.1243. [ DOI ] [ PubMed ] [ Google Scholar ]
- Mataga N, Nagai N, Hensch TK. Permissive proteolytic activity for visual cortical plasticity. Proc. Natl. Acad. Sci. USA. 2002;99:7717–7721. doi: 10.1073/pnas.102088899. [ DOI ] [ PMC free article ] [ PubMed ] [ Google Scholar ]
- Maya Vetencourt JF, Sale A, Viegi A, Baroncelli L, De Pasquale R, O’Leary OF, Castrén E, Maffei L. The antidepressant fluoxetine restores plasticity in the adult visual cortex. Science. 2008;320:385–388. doi: 10.1126/science.1150516. [ DOI ] [ PubMed ] [ Google Scholar ]
- McCurry CL, Shepherd JD, Tropea D, Wang KH, Bear MF, Sur M. Loss of Arc renders the visual cortex impervious to the effects of sensory experience or deprivation. Nat. Neurosci. 2010;13:450–457. doi: 10.1038/nn.2508. [ DOI ] [ PMC free article ] [ PubMed ] [ Google Scholar ]
- McGee AW, Yang Y, Fischer QS, Daw NW, Strittmatter SM. Experience-driven plasticity of visual cortex limited by myelin and Nogo receptor. Science. 2005;309:2222–2226. doi: 10.1126/science.1114362. [ DOI ] [ PMC free article ] [ PubMed ] [ Google Scholar ]
- Mellios N, Sugihara H, Castro J, Banerjee A, Le C, Kumar A, Crawford B, Strathmann J, Tropea D, Levine SS, et al. miR-132, an experience-dependent microRNA, is essential for visual cortex plasticity. Nat. Neurosci. 2011;14:1240–1242. doi: 10.1038/nn.2909. [ DOI ] [ PMC free article ] [ PubMed ] [ Google Scholar ]
- Miller KD, Keller JB, Stryker MP. Ocular dominance column development: analysis and simulation. Science. 1989;245:605–615. doi: 10.1126/science.2762813. [ DOI ] [ PubMed ] [ Google Scholar ]
- Miller EW, Lin JY, Frady EP, Steinbach PA, Kristan WB, Jr, Tsien RY. Optically monitoring voltage in neurons by photo-induced electron transfer through molecular wires. Proc. Natl. Acad. Sci. USA. 2012;109:2114–2119. doi: 10.1073/pnas.1120694109. [ DOI ] [ PMC free article ] [ PubMed ] [ Google Scholar ]
- Mioche L, Singer W. Chronic recordings from single sites of kitten striate cortex during experience-dependent modifications of receptive-field properties. J. Neurophysiol. 1989;62:185–197. doi: 10.1152/jn.1989.62.1.185. [ DOI ] [ PubMed ] [ Google Scholar ]
- Morales B, Choi SY, Kirkwood A. Dark rearing alters the development of GABAergic transmission in visual cortex. J. Neurosci. 2002;22:8084–8090. doi: 10.1523/JNEUROSCI.22-18-08084.2002. [ DOI ] [ PMC free article ] [ PubMed ] [ Google Scholar ]
- Morishita H, Miwa JM, Heintz N, Hensch TK. Lynx1, a cholinergic brake, limits plasticity in adult visual cortex. Science. 2010;330:1238–1240. doi: 10.1126/science.1195320. [ DOI ] [ PMC free article ] [ PubMed ] [ Google Scholar ]
- Mrsic-Flogel TD, Hofer SB, Ohki K, Reid RC, Bonhoeffer T, Hübener M. Homeostatic regulation of eye-specific responses in visual cortex during ocular dominance plasticity. Neuron. 2007;54:961–972. doi: 10.1016/j.neuron.2007.05.028. [ DOI ] [ PubMed ] [ Google Scholar ]
- Niell CM, Stryker MP. Highly selective receptive fields in mouse visual cortex. J. Neurosci. 2008;28:7520–7536. doi: 10.1523/JNEUROSCI.0623-08.2008. [ DOI ] [ PMC free article ] [ PubMed ] [ Google Scholar ]
- O’Leary DD, Chou SJ, Sahara S. Area patterning of the mammalian cortex. Neuron. 2007;56:252–269. doi: 10.1016/j.neuron.2007.10.010. [ DOI ] [ PubMed ] [ Google Scholar ]
- Ohki K, Chung S, Ch’ng YH, Kara P, Reid RC. Functional imaging with cellular resolution reveals precise micro-architecture in visual cortex. Nature. 2005;433:597–603. doi: 10.1038/nature03274. [ DOI ] [ PubMed ] [ Google Scholar ]
- Oray S, Majewska A, Sur M. Dendritic spine dynamics are regulated by monocular deprivation and extracellular matrix degradation. Neuron. 2004;44:1021–1030. doi: 10.1016/j.neuron.2004.12.001. [ DOI ] [ PubMed ] [ Google Scholar ]
- Priebe NJ, Ferster D. Mechanisms of neuronal computation in mammalian visual cortex. Neuron. 2012;75:194–208. doi: 10.1016/j.neuron.2012.06.011. this issue. [ DOI ] [ PMC free article ] [ PubMed ] [ Google Scholar ]
- Prusky GT, Douglas RM. Developmental plasticity of mouse visual acuity. Eur. J. Neurosci. 2003;17:167–173. doi: 10.1046/j.1460-9568.2003.02420.x. [ DOI ] [ PubMed ] [ Google Scholar ]
- Rakic P. Prenatal genesis of connections subserving ocular dominance in the rhesus monkey. Nature. 1976;261:467–471. doi: 10.1038/261467a0. [ DOI ] [ PubMed ] [ Google Scholar ]
- Ramoa AS, Paradiso MA, Freeman RD. Blockade of intracortical inhibition in kitten striate cortex: effects on receptive field properties and associated loss of ocular dominance plasticity. Exp. Brain Res. 1988;73:285–296. doi: 10.1007/BF00248220. [ DOI ] [ PubMed ] [ Google Scholar ]
- Ranson A, Cheetham CE, Fox K, Sengpiel F. Homeostatic plasticity mechanisms are required for juvenile, but not adult, ocular dominance plasticity. Proc. Natl. Acad. Sci. USA. 2012;109:1311–1316. doi: 10.1073/pnas.1112204109. [ DOI ] [ PMC free article ] [ PubMed ] [ Google Scholar ]
- Rao Y, Fischer QS, Yang Y, McKnight GS, LaRue A, Daw NW. Reduced ocular dominance plasticity and long-term potentiation in the developing visual cortex of protein kinase A RII alpha mutant mice. Eur. J. Neurosci. 2004;20:837–842. doi: 10.1111/j.1460-9568.2004.03499.x. [ DOI ] [ PubMed ] [ Google Scholar ]
- Reid RC. From functional architecture to functional connectomics. Neuron. 2012;75:209–217. doi: 10.1016/j.neuron.2012.06.031. this issue. [ DOI ] [ PMC free article ] [ PubMed ] [ Google Scholar ]
- Reiter HO, Stryker MP. Neural plasticity without postsynaptic action potentials: less-active inputs become dominant when kitten visual cortical cells are pharmacologically inhibited. Proc. Natl. Acad. Sci. USA. 1988;85:3623–3627. doi: 10.1073/pnas.85.10.3623. [ DOI ] [ PMC free article ] [ PubMed ] [ Google Scholar ]
- Reiter HO, Waitzman DM, Stryker MP. Cortical activity blockade prevents ocular dominance plasticity in the kitten visual cortex. Exp. Brain Res. 1986;65:182–188. doi: 10.1007/BF00243841. [ DOI ] [ PubMed ] [ Google Scholar ]
- Riesen AH. Stimulation as a requirement for growth and function in behavioral development. In: Fiske DW, Maddi SR, editors. Functions of Varied Experience. Homewood, Illinois: Dorsey Press; 1961. [ Google Scholar ]
- Ringach DL. On the origin of the functional architecture of the cortex. PLoS ONE. 2007;2:e251. doi: 10.1371/journal.pone.0000251. [ DOI ] [ PMC free article ] [ PubMed ] [ Google Scholar ]
- Roberts EB, Meredith MA, Ramoa AS. Suppression of NMDA receptor function using antisense DNA block ocular dominance plasticity while preserving visual responses. J. Neurophysiol. 1998;80:1021–1032. doi: 10.1152/jn.1998.80.3.1021. [ DOI ] [ PubMed ] [ Google Scholar ]
- Rochefort NL, Narushima M, Grienberger C, Marandi N, Hill DN, Konnerth A. Development of direction selectivity in mouse cortical neurons. Neuron. 2011;71:425–432. doi: 10.1016/j.neuron.2011.06.013. [ DOI ] [ PubMed ] [ Google Scholar ]
- Rogan SC, Roth BL. Remote control of neuronal signaling. Pharmacol. Rev. 2011;63:291–315. doi: 10.1124/pr.110.003020. [ DOI ] [ PMC free article ] [ PubMed ] [ Google Scholar ]
- Sato M, Stryker MP. Distinctive features of adult ocular dominance plasticity. J. Neurosci. 2008;28:10278–10286. doi: 10.1523/JNEUROSCI.2451-08.2008. [ DOI ] [ PMC free article ] [ PubMed ] [ Google Scholar ]
- Sato M, Stryker MP. Genomic imprinting of experience-dependent cortical plasticity by the ubiquitin ligase gene Ube3a. Proc. Natl. Acad. Sci. USA. 2010;107:5611–5616. doi: 10.1073/pnas.1001281107. [ DOI ] [ PMC free article ] [ PubMed ] [ Google Scholar ]
- Sato TK, Nauhaus I, Carandini M. Traveling waves in visual cortex. Neuron. 2012;75:218–229. doi: 10.1016/j.neuron.2012.06.029. this issue. [ DOI ] [ PubMed ] [ Google Scholar ]
- Sawtell NB, Frenkel MY, Philpot BD, Nakazawa K, Tonegawa S, Bear MF. NMDA receptor-dependent ocular dominance plasticity in adult visual cortex. Neuron. 2003;38:977–985. doi: 10.1016/s0896-6273(03)00323-4. [ DOI ] [ PubMed ] [ Google Scholar ]
- Shaw C, Cynader M. Disruption of cortical activity prevents ocular dominance changes in monocularly deprived kittens. Nature. 1984;308:731–734. doi: 10.1038/308731a0. [ DOI ] [ PubMed ] [ Google Scholar ]
- Sherk H, Stryker MP. Quantitative study of cortical orientation selectivity in visually inexperienced kitten. J. Neurophysiol. 1976;39:63–70. doi: 10.1152/jn.1976.39.1.63. [ DOI ] [ PubMed ] [ Google Scholar ]
- Sieghart W. Structure and pharmacology of gamma-aminobutyric acidA receptor subtypes. Pharmacol. Rev. 1995;47:181–234. [ PubMed ] [ Google Scholar ]
- Smith SL, Trachtenberg JT. Experience-dependent binocular competition in the visual cortex begins at eye opening. Nat. Neurosci. 2007;10:370–375. doi: 10.1038/nn1844. [ DOI ] [ PubMed ] [ Google Scholar ]
- Sohal VS, Zhang F, Yizhar O, Deisseroth K. Parvalbumin neurons and gamma rhythms enhance cortical circuit performance. Nature. 2009;459:698–702. doi: 10.1038/nature07991. [ DOI ] [ PMC free article ] [ PubMed ] [ Google Scholar ]
- Southwell DG, Froemke RC, Alvarez-Buylla A, Stryker MP, Gandhi SP. Cortical plasticity induced by inhibitory neuron transplantation. Science. 2010;327:1145–1148. doi: 10.1126/science.1183962. [ DOI ] [ PMC free article ] [ PubMed ] [ Google Scholar ]
- Stellwagen D, Malenka RC. Synaptic scaling mediated by glial TNF-alpha. Nature. 2006;440:1054–1059. doi: 10.1038/nature04671. [ DOI ] [ PubMed ] [ Google Scholar ]
- Stent GS. A physiological mechanism for Hebb’s postulate of learning. Proc. Natl. Acad. Sci. USA. 1973;70:997–1001. doi: 10.1073/pnas.70.4.997. [ DOI ] [ PMC free article ] [ PubMed ] [ Google Scholar ]
- Steward O, Worley PF. Selective targeting of newly synthesized Arc mRNA to active synapses requires NMDA receptor activation. Neuron. 2001;30:227–240. doi: 10.1016/s0896-6273(01)00275-6. [ DOI ] [ PubMed ] [ Google Scholar ]
- Stryker MP, Harris WA. Binocular impulse blockade prevents the formation of ocular dominance columns in cat visual cortex. J. Neurosci. 1986;6:2117–2133. doi: 10.1523/JNEUROSCI.06-08-02117.1986. [ DOI ] [ PMC free article ] [ PubMed ] [ Google Scholar ]
- Stryker MP, Sherk H. Modification of cortical orientation selectivity in the cat by restricted visual experience: a reexamination. Science. 1975;190:904–906. doi: 10.1126/science.1188372. [ DOI ] [ PubMed ] [ Google Scholar ]
- Stryker MP, Sherk H, Leventhal AG, Hirsch HV. Physiological consequences for the cat’s visual cortex of effectively restricting early visual experience with oriented contours. J. Neurophysiol. 1978;41:896–909. doi: 10.1152/jn.1978.41.4.896. [ DOI ] [ PubMed ] [ Google Scholar ]
- Sugiyama S, Di Nardo AA, Aizawa S, Matsuo I, Volovitch M, Prochiantz A, Hensch TK. Experience-dependent transfer of Otx2 homeoprotein into the visual cortex activates postnatal plasticity. Cell. 2008;134:508–520. doi: 10.1016/j.cell.2008.05.054. [ DOI ] [ PubMed ] [ Google Scholar ]
- Syken J, Grandpre T, Kanold PO, Shatz CJ. PirB restricts ocular-dominance plasticity in visual cortex. Science. 2006;313:1795–1800. doi: 10.1126/science.1128232. [ DOI ] [ PubMed ] [ Google Scholar ]
- Taha S, Stryker MP. Rapid ocular dominance plasticity requires cortical but not geniculate protein synthesis. Neuron. 2002;34:425–436. doi: 10.1016/s0896-6273(02)00673-6. [ DOI ] [ PubMed ] [ Google Scholar ]
- Taha S, Hanover JL, Silva AJ, Stryker MP. Autophosphorylation of alphaCaMKII is required for ocular dominance plasticity. Neuron. 2002;36:483–491. doi: 10.1016/s0896-6273(02)00966-2. [ DOI ] [ PubMed ] [ Google Scholar ]
- Tamamaki N, Yanagawa Y, Tomioka R, Miyazaki J, Obata K, Kaneko T. Green fluorescent protein expression and colocalization with calretinin, parvalbumin, and somatostatin in the GAD67-GFP knock-in mouse. J. Comp. Neurol. 2003;467:60–79. doi: 10.1002/cne.10905. [ DOI ] [ PubMed ] [ Google Scholar ]
- Taniguchi H, He M, Wu P, Kim S, Paik R, Sugino K, Kvitsiani D, Fu Y, Lu J, Lin Y, et al. A resource of Cre driver lines for genetic targeting of GABAergic neurons in cerebral cortex. Neuron. 2011;71:995–1013. doi: 10.1016/j.neuron.2011.07.026. [ DOI ] [ PMC free article ] [ PubMed ] [ Google Scholar ]
- Tian N, Copenhagen DR. Visual stimulation is required for refinement of ON and OFF pathways in postnatal retina. Neuron. 2003;39:85–96. doi: 10.1016/s0896-6273(03)00389-1. [ DOI ] [ PubMed ] [ Google Scholar ]
- Tian L, Hires SA, Mao T, Huber D, Chiappe ME, Chalasani SH, Petreanu L, Akerboom J, McKinney SA, Schreiter ER, et al. Imaging neural activity in worms, flies and mice with improved GCaMP calcium indicators. Nat. Methods. 2009;6:875–881. doi: 10.1038/nmeth.1398. [ DOI ] [ PMC free article ] [ PubMed ] [ Google Scholar ]
- Tognini P, Putignano E, Coatti A, Pizzorusso T. Experience-dependent expression of miR-132 regulates ocular dominance plasticity. Nat. Neurosci. 2011;14:1237–1239. doi: 10.1038/nn.2920. [ DOI ] [ PMC free article ] [ PubMed ] [ Google Scholar ]
- Toyoizumi T, Miller KD. Equalization of ocular dominance columns induced by an activity-dependent learning rule and the maturation of inhibition. J. Neurosci. 2009;29:6514–6525. doi: 10.1523/JNEUROSCI.0492-08.2009. [ DOI ] [ PMC free article ] [ PubMed ] [ Google Scholar ]
- Trachtenberg JT, Stryker MP. Rapid anatomical plasticity of horizontal connections in the developing visual cortex. J. Neurosci. 2001;21:3476–3482. doi: 10.1523/JNEUROSCI.21-10-03476.2001. [ DOI ] [ PMC free article ] [ PubMed ] [ Google Scholar ]
- Trachtenberg JT, Trepel C, Stryker MP. Rapid extragranular plasticity in the absence of thalamocortical plasticity in the developing primary visual cortex. Science. 2000;287:2029–2032. doi: 10.1126/science.287.5460.2029. [ DOI ] [ PMC free article ] [ PubMed ] [ Google Scholar ]
- Tsien RY. Nobel lecture: constructing and exploiting the fluorescent protein paintbox. Integr Biol (Camb) 2010;2:77–93. doi: 10.1039/b926500g. [ DOI ] [ PubMed ] [ Google Scholar ]
- Turrigiano GG, Nelson SB. Homeostatic plasticity in the developing nervous system. Nat. Rev. Neurosci. 2004;5:97–107. doi: 10.1038/nrn1327. [ DOI ] [ PubMed ] [ Google Scholar ]
- van Versendaal D, Rajendran R, Saiepour MH, Klooster J, Smit-Rigter L, Sommeijer JP, De Zeeuw CI, Hofer SB, Heimel JA, Levelt CN. Elimination of inhibitory synapses is a major component of adult ocular dominance plasticity. Neuron. 2012;74:374–383. doi: 10.1016/j.neuron.2012.03.015. [ DOI ] [ PubMed ] [ Google Scholar ]
- Wagor E, Mangini NJ, Pearlman AL. Retinotopic organization of striate and extrastriate visual cortex in the mouse. J. Comp. Neurol. 1980;193:187–202. doi: 10.1002/cne.901930113. [ DOI ] [ PubMed ] [ Google Scholar ]
- Wang BS, Sarnaik R, Cang J. Critical period plasticity matches binocular orientation preference in the visual cortex. Neuron. 2010;65:246–256. doi: 10.1016/j.neuron.2010.01.002. [ DOI ] [ PMC free article ] [ PubMed ] [ Google Scholar ]
- Wehr M, Zador AM. Balanced inhibition underlies tuning and sharpens spike timing in auditory cortex. Nature. 2003;426:442–446. doi: 10.1038/nature02116. [ DOI ] [ PubMed ] [ Google Scholar ]
- Weliky M, Katz LC. Correlational structure of spontaneous neuronal activity in the developing lateral geniculate nucleus in vivo. Science. 1999;285:599–604. doi: 10.1126/science.285.5427.599. [ DOI ] [ PubMed ] [ Google Scholar ]
- White LE, Coppola DM, Fitzpatrick D. The contribution of sensory experience to the maturation of orientation selectivity in ferret visual cortex. Nature. 2001;411:1049–1052. doi: 10.1038/35082568. [ DOI ] [ PubMed ] [ Google Scholar ]
- Wickersham IR, Lyon DC, Barnard RJ, Mori T, Finke S, Conzelmann KK, Young JA, Callaway EM. Monosynaptic restriction of transsynaptic tracing from single, genetically targeted neurons. Neuron. 2007;53:639–647. doi: 10.1016/j.neuron.2007.01.033. [ DOI ] [ PMC free article ] [ PubMed ] [ Google Scholar ]
- Wiesel TN, Hubel DH. Effects of Visual Deprivation on Morphology and Physiology of Cells in the Cats Lateral Geniculate Body. J. Neurophysiol. 1963a;26:978–993. doi: 10.1152/jn.1963.26.6.978. [ DOI ] [ PubMed ] [ Google Scholar ]
- Wiesel TN, Hubel DH. Single-Cell Responses in Striate Cortex of Kittens Deprived of Vision in One Eye. J. Neurophysiol. 1963b;26:1003–1017. doi: 10.1152/jn.1963.26.6.1003. [ DOI ] [ PubMed ] [ Google Scholar ]
- Wiesel TN, Hubel DH. Comparison of the effects of unilateral and bilateral eye closure on cortical unit responses in kittens. J. Neurophysiol. 1965;28:1029–1040. doi: 10.1152/jn.1965.28.6.1029. [ DOI ] [ PubMed ] [ Google Scholar ]
- Wiesel TN, Hubel DH. Ordered arrangement of orientation columns in monkeys lacking visual experience. J. Comp. Neurol. 1974;158:307–318. doi: 10.1002/cne.901580306. [ DOI ] [ PubMed ] [ Google Scholar ]
- Wiesel TN, Hubel DH, Lam DM. Autoradiographic demonstration of ocular-dominance columns in the monkey striate cortex by means of transneuronal transport. Brain Res. 1974;79:273–279. doi: 10.1016/0006-8993(74)90416-8. [ DOI ] [ PubMed ] [ Google Scholar ]
- Willshaw DJ, von der Malsburg C. How patterned neural connections can be set up by self-organization. Proc. R. Soc. Lond. B Biol. Sci. 1976;194:431–445. doi: 10.1098/rspb.1976.0087. [ DOI ] [ PubMed ] [ Google Scholar ]
- Wong RO, Meister M, Shatz CJ. Transient period of correlated bursting activity during development of the mammalian retina. Neuron. 1993;11:923–938. doi: 10.1016/0896-6273(93)90122-8. [ DOI ] [ PubMed ] [ Google Scholar ]
- Yang Y, Fischer QS, Zhang Y, Baumgärtel K, Mansuy IM, Daw NW. Reversible blockade of experience-dependent plasticity by calcineurin in mouse visual cortex. Nat. Neurosci. 2005;8:791–796. doi: 10.1038/nn1464. [ DOI ] [ PubMed ] [ Google Scholar ]
- Yao H, Dan Y. Synaptic learning rules, cortical circuits, and visual function. Neuroscientist. 2005;11:206–216. doi: 10.1177/1073858404272404. [ DOI ] [ PubMed ] [ Google Scholar ]
- Yazaki-Sugiyama Y, Kang S, Câteau H, Fukai T, Hensch TK. Bidirectional plasticity in fast-spiking GABA circuits by visual experience. Nature. 2009;462:218–221. doi: 10.1038/nature08485. [ DOI ] [ PubMed ] [ Google Scholar ]
- Yoon BJ, Smith GB, Heynen AJ, Neve RL, Bear MF. Essential role for a long-term depression mechanism in ocular dominance plasticity. Proc. Natl. Acad. Sci. USA. 2009;106:9860–9865. doi: 10.1073/pnas.0901305106. [ DOI ] [ PMC free article ] [ PubMed ] [ Google Scholar ]
- Zafra F, Hengerer B, Leibrock J, Thoenen H, Lindholm D. Activity dependent regulation of BDNF and NGF mRNAs in the rat hippocampus is mediated by non-NMDA glutamate receptors. EMBO J. 1990;9:3545–3550. doi: 10.1002/j.1460-2075.1990.tb07564.x. [ DOI ] [ PMC free article ] [ PubMed ] [ Google Scholar ]
- View on publisher site
- PDF (3.5 MB)
- Collections
Similar articles
Cited by other articles, links to ncbi databases.
- Download .nbib .nbib
- Format: AMA APA MLA NLM
Add to Collections

IMAGES
VIDEO